- Search Menu
- Advance Articles
- Collections
- Focus Collections
- Teaching Tools in Plant Biology
- Browse by cover
- High-Impact Research
- Author Guidelines
- Quick and Simple Author Support
- Focus Issues Call for Papers
- Submission Site
- Open Access Options
- Self-Archiving Policy
- Why Publish with Us?
- About The Plant Cell
- About The American Society of Plant Biologists
- Editorial Board
- Advertising & Corporate Services
- Journals on Oxford Academic
- Books on Oxford Academic
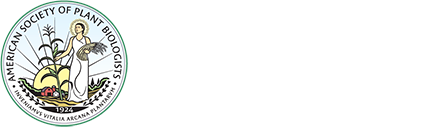

Article Contents
- < Previous
Why Study Plants?
- Article contents
- Figures & tables
- Supplementary Data
Why Study Plants?, The Plant Cell , Volume 21, Issue 10, October 2009, No Pagination Specified, https://doi.org/10.1105/tpc.109.tt1009
- Permissions Icon Permissions
Plants provide us with oxygen, food, fuel and fiber. Among other reasons, scientists study plants to improve and secure the food supply for an increasing world population, identify new sources of bioactive compounds and medicines, improve fiber production and identify sources of biofuels and biorenewable resources. This lecture is designed for a general audience or first year university students - no prior knowledge is assumed.
Click HERE to access Teaching Tool Components
Supplementary data
Email alerts, citing articles via.
- Recommend to Your Librarian
- Advertising & Corporate Services
- Awards & Funding
- Plant Science Today
- Plant Biology Meeting
- Meeting Management Services
- Plant Science Research Weekly
- Taproot: A Plantae Podcast
Affiliations
- Online ISSN 1532-298X
- Print ISSN 1040-4651
- Copyright © 2024 American Society of Plant Biologists
- About Oxford Academic
- Publish journals with us
- University press partners
- What we publish
- New features
- Open access
- Institutional account management
- Rights and permissions
- Get help with access
- Accessibility
- Advertising
- Media enquiries
- Oxford University Press
- Oxford Languages
- University of Oxford
Oxford University Press is a department of the University of Oxford. It furthers the University's objective of excellence in research, scholarship, and education by publishing worldwide
- Copyright © 2024 Oxford University Press
- Cookie settings
- Cookie policy
- Privacy policy
- Legal notice
This Feature Is Available To Subscribers Only
Sign In or Create an Account
This PDF is available to Subscribers Only
For full access to this pdf, sign in to an existing account, or purchase an annual subscription.
- Review Paper
- Open access
- Published: 28 April 2021
The relationship between plant growth and water consumption: a history from the classical four elements to modern stable isotopes
- Oliver Brendel ORCID: orcid.org/0000-0003-3252-0273 1
Annals of Forest Science volume 78 , Article number: 47 ( 2021 ) Cite this article
32k Accesses
18 Citations
15 Altmetric
Metrics details
A Correction to this article was published on 17 June 2021
This article has been updated
Key message
The history of the relationship between plant growth and water consumption is retraced by following the progression of scientific thought through the centuries: from a purely philosophical question, to conceptual and methodological developments, towards a research interest in plant functioning and the interaction with the environment.
The relationship between plant growth and water consumption has for a long time occupied the minds of philosophers and natural scientists. The ratio between biomass accumulation and water consumption is known as water use efficiency and is widely relevant today in fields as diverse as plant improvement, forest ecology and climate change. Defined at scales varying from single leaf physiology to whole plants, it shows how botanical investigations changed through time, generally in tandem with developing disciplines and improving methods. The history started as a purely philosophical question by Greek philosophers of how plants grow, progressed through thought and actual experiments, towards an interest in the functioning of plants and the relationship to the environment.
This article retraces this history by following the progression of scientific questions posed through the centuries, and presents not only the main methodological and conceptual developments on biomass growth and transpiration but also the development of the carbon isotopic method of estimation. The history of research on photosynthesis is only touched briefly, but the development of research on transpiration and stomatal conductance is presented with more detail.
Research on water use efficiency, following a path from the whole plant to leaf-level functioning, was strongly involved in the historical development of the discipline of plant ecophysiology and is still a very active research field across nearly all levels of botanical research.
1 Introduction
The ratio of biomass accumulation per unit water consumption is known today as water use efficiency (WUE) and is widely relevant to agriculture (e.g. Blum 2009 ; Tallec et al. 2013 ; Vadez et al. 2014 ), to forest ecology (e.g. Linares and Camarero 2012 ; Lévesque et al. 2014 ) and in the context of global climate change (Cernusak et al. 2019 ). This ratio can be defined at various levels, from the physiological functioning of a leaf to the whole plant and at the ecosystem level. This historical review starts at the whole plant level, where WUE can be simply measured by quantifying the amount of water given to a plant and the plant’s increase in biomass during the experiment. The ratio of biomass produced divided by the cumulative water lost during growth is termed whole plant transpiration efficiency (TE= biomass produced/water lost). Historically, the ratio has also been calculated in its inverted form (water lost/biomass produced) and various terms have been used to denote these ratios (see Box 1). As knowledge, concepts and technology advanced, it became desirable to measure TE also at the leaf level, where it is defined either as the ratio of net CO 2 assimilation rate to transpiration (or to the stomatal conductance for water vapour). Therefore, some history of the two leaf-level components of WUE is included here. Numerous articles have been published on the history of the development of research on photosynthesis, and other than the reviews cited in this article, the publications by Govindjee are notable, especially Govindjee and Krogmann ( 2004 ), as they include a long list of other writings on the history of photosynthesis. On the other hand, little has been written about the history of research on transpiration and stomatal conductance. Notable is Brown ( 2013 ), who wrote specifically on the cohesion-tension theory of the rise of sap in trees, including many writings from the late nineteenth century. Consequently, here, photosynthesis research is only broached briefly, whereas transpiration research is more detailed.
As the development of the research on WUE spans a very long period, starting with Greek philosophers, publications are in several languages. Classical writings were in Greek or in Latin, and for these translations are available. However, from the mid-seventeenth century onwards, national languages were more and more used, which can be seen in the number of French- and German-language publications. This review is also a tribute to these nowadays less known seventeenth, eighteenth and nineteenth century French and German natural philosophers and their contribution to the development of the science of plant ecophysiology. Also, towards the beginning of the twentieth century, publications became too numerous to allow a comprehensive review; thus, the author focussed on the use of the carbon stable isotopes methodology and on tree ecology.
Box 1 Short history of names for whole plant transpiration efficiency (TE)
2 what is plant matter made of.
Various Greek philosophers were interested in how substances can change from one thing into another. Thales (624–c. 546 BC) thought that all things come from water, whereas Anaximenes argued that “pneuma” (air) should be the basis of all things (Egerton 2001a ). These assertions were the basis of more than 2000 years of philosophical dispute.
In “De Causis Plantarum”, Theophrastos (371–287 BC) assumed that plants draw nutrition, which consisted of varying amounts of the four elementary humours, from the earth through their roots (Morton 1981 ). Some centuries later, in a Christian work translated in 400 AD from Greek into Latin and known as “Pseudo-Clement’s Recognitions”, an apparent thought experiment was described to “prove that nothing is supplied to seeds from the substance of the earth, but that they are entirely derived from the element of water and the spirit (spiritus) that is in it” (Egerton 2004c ). The author of this thought experiment suggested putting earth into big barrels, growing herbaceous plants in it for several years, then harvesting them and weighing them. His hypothesis was that the weight of the earth would not have changed, and the author used this as an argument that the vegetation biomass could have come only from water. This thought experiment revealed a progress in scientific thinking because the question was posed more precisely than before. It stood out at a time when botany mainly consisted of naming plants and “theoretical botany effectually went out of existence” (Morton 1981 ).
It appears that the question of how plant matter is produced was not pursued in Roman or Arabic writings, which were more concerned with agricultural (the former) and medical (the latter) aspects of plant sciences (Egerton 2001b , 2002 ). Not until the High Middle Ages was a renewed interest shown in plant growth. Adelard of Bath, a twelfth century English natural philosopher, devoted the first four chapters of “Questiones Naturales” (c. 1130–1140; Morton 1981 ) to the question of what plant matter is made of. He argued, within the concepts of the four elements theory, “by just as much as water differs from earth, by so much does it afford less nourishment to roots, I mean than earth does”, clearly being in favour of earth as the source for plant nourishment. His arguments were only theoretical and speculative.
A major step occurred in botanical sciences between the fifteenth and sixteenth centuries; scholars began making experiments to test antique and medieval hypotheses against observations in nature (Egerton 2003 ). In the mid-fifteenth century, and probably related to the translation and printing of the botanical books by Theophrastus (Morton 1981 ), the thought experiment from “Recognitions...” was taken up by Nicholas of Cusa in the fourth part of his “Idiota de mente”, “De staticis experiments”. At a time when the naming of plants for pharmacology was the major interest of savants, he proposed experimental investigations. Nicholas of Cusa described the same thought experiment as did Pseudo-Clement’s Recognitions ; he concluded similarly that “the collected herbs have weight mainly from water” (1450; translation into English by Hopkins 1996 ). Cusa additionally suggested that the plants should be burned at the end of the experiment and the ash weight be taken into account. It is not clear whether the thought experiment was ever physically done.
In the sixteenth century, botanical science began to separate from medical sciences, with the establishment of lectureships in universities (e.g. Padua in 1533) and the establishment of botanical gardens (Egerton 2003 ). The bases existed for advancing science in the seventeenth century of Enlightenment. Francis Bacon, an influential philosopher of his time, conducted a series of plant growth experiments which are reported in his “de Augmentis Scientiarum” (1623; Spedding et al. 1900 ). Bacon discovered that some plants sprouted more quickly in water than in soil (Egerton 2004b ). He concluded that “for nourishment the water is almost all in all, and that the earth doth but keep the plant upright, and save it from over-heat and over-cold” (Hershey 2003 ), thus still upholding the theory proposed by Thales and Nicholas of Cusa. In “The History of the Propagation and Improvement of Vegetable”, Robert Sharrock ( 1660 ) reported that some plants both rooted and grew entirely in water. Although he noted different amounts of transpiration over time, he did not discuss this in relation to plant growth.
In 1662, Johannes Baptista van Helmont published his now-famous willow experiments (van Helmont 1662 ). This may be the first report of an experiment that was based on the thought experiment of Nicholas of Cusa (Hershey 2003 ) with the minor differences of beginning with dried soil and not using herbaceous plants, but rather a willow tree. After weighing the soil, he irrigated it with rain water and planted the weighed stem of a willow tree. The experiment ran for 5 years. At the end, the tree was weighed again, as was the dried soil. He found the soil weighed about 2 ounces less than at the beginning of the experiment, whereas 164 pounds of wood, bark and roots was produced. He concluded that the organic matter could only have come out of the water. Helmont was unaware of the existence of carbon dioxide, but he did know of “gas sylvestre”. He also knew that burning oak charcoal would produce nearly the same amount of gas sylvestre and ash. However, he did not connect this information with the plant growth he had observed (Hershey 2003 ). Robert Boyle published similar experiments in “The sceptical Chymist” (Boyle 1661 ). Boyle claimed that he had done his experiments before he knew of Helmont’s (Egerton 2004c ), although he discussed Helmont’s results and arguments in detail in his book. Boyle doubted the direct transformation of water into plant matter. He admitted, however, that it might be possible that other substances contained in the water could generate new matter (Boyle 1661 ). In the 1660’s, Edme Mariotte also criticised van Helmont’s theory that water alone constituted the only element to produce plant matter. He thought similarly to Boyle that elements in the water could contribute to the plant matter. He also showed that nitrogen compounds were important for plant growth (Bugler 1950 ).
John Woodward, in his “Some Thoughts and Experiments Concerning Vegetation” (Woodward 1699 ), took up again the question of what comprised the source of plant growth. Woodward criticised Helmont’s and Boyle’s experiments, mainly on the precision of weighing the dry soil before and after the experiment, but also the contamination of the irrigation water by terrestrial vegetable or mineral matter. Consequently, he developed a series of hydroponics experiments, where by growing plants in sealed vials, in different types of water and weighing them regularly over the same time period, he could calculate how much biomass was gained over a set time period. He was able to draw a series of conclusions from these experiments by calculating the ratio of water lost to plant mass gained in the same period of time, thereby calculating the inverse of transpiration efficiency. This was probably the first time that the inverse of transpiration efficiency was calculated using experimental data. He showed that 50 to 700 times as much water was lost than biomass gained. He also reported that plants grown in water containing more terrestrial matter grew more and with less water consumed. From these observations, he concluded that water serves only as a vehicle for the terrestrial matter that forms vegetables and that vegetable matter is not formed out of water. He is still remembered more for his geological publications (Porter 1979 ) than for his contributions to botany (Stanhill 1986 ).
In his “history of ecology” series, Egerton ( 2004c ) nicely sums this period thusly: “each of these authors (Bacon, Boyle, Helmont, Sharrock) built upon the work of his predecessors and improved somewhat the understanding of plant growth and how to study it. However, they still fell short of a basic understanding of plant growth. Before that could be achieved, chemists would have to identify the gases in the air”. This series of studies shows that from the end of the seventeenth century onwards, experiments replaced speculation (Morton 1981 ), in botany as well as in many other areas of science.
From the end of the seventeenth century, the question of how plants grow was still unresolved, although it was known that nutrients were conducted from the roots in the ascending sap to the leaves. A major improvement in the understanding of how transpiration and its variations work was the discovery of cells by Robert Hooke towards the middle of the seventeenth century (Egerton 2005 ) and subsequently the discovery of stomata on leaf surfaces. One of the first to describe stomata may have been Malpighi in “Anatomy of Plants” (Malpighi 1675 in Möbius 1901 ). Based on Malpighi’s and Grew’s ( 1682 ) studies, John Ray suggested in “Historia Plantarum” (Ray 1686 in Lazenby 1995 ) that the apertures in the leaves, when open, would give off either breath or liquid. Ray may have been the first to have connected stomata with transpiration. He also suggested that the loss of water by evaporation is compensated constantly by water from the stem, and thus transpiration results from a constant water flux. He also observed that sap ascends the stems of trees in sap-bearing vessels which do not contain valves. He did, however, admit that it cannot be capillary forces that make water go up tall trees.
Ideas on photosynthesis developed slowly from the middle of the seventeenth century onwards. Malpighi ( 1675 ) suggested that leaves produce (“concoct and prepare”) the food of plants and from leaves this food passes to all parts of the plant. Similarly, Claude Perrault in “Essais de Physique” (Perrault 1680 ) defended the hypothesis that the root acts as the mouth of the plant and that the leaves serve to prepare the food arriving with the sap from the root so that it can be used in the rest of the plant. John Ray in “History Plantarum” (Ray 1686 in Lazenby 1995 ) concurs with this, however adding in “The wisdom of God” (Ray 1691 in Lazenby 1995 ) that “not only that which ascends from the Root, but that which they take in from without, from the Dew, moist Air, and Rain”. He also thought that light could play a role in this preparation of the plant sap. At this time, most authors (Malpighi, Perrault, Mariotte, Ray) knew about the circulation of sap, up as well as down, and that leaves served somehow to transform the upcoming sap into food for the plant.
In 1770 , Lavoisier published “Sur la nature de l’eau” (“On the nature of water”, translation by the author) and reviewed the literature on the possibility of water changing into earth to nourish plants. Lavoisier cited the Van Helmont experiment and later works which tested Van Helmont’s idea by growing plants in water (e.g. Boyle, however he did not cite Woodward). He was critical of the idea that it could be a transformation of water that would constitute plant material. This was based mainly on experiments by himself and others, showing even distilled water would contain traces of “soil”. However, he also defended the idea, based mainly on Charles Bonnet’s observations, that leaves absorb vapours from the atmosphere that contribute to plant growth.
Helmont had coined the term “gaz” in the mid-seventeenth century and had been able to distinguish different gazes from air (Egerton 2004a ). It was only in the middle of the eighteenth century that gases were studied in the laboratory and several observations by different researchers would finally lead to an understanding of respiration and photosynthesis (Tomic et al. 2005 ; Nickelsen 2007 ). Richard Bradley seems to be one of the first to clearly state (in letters from 1721 to 1724) that plant nourishment can be drawn from the air. Hales ( 1727 ) agreed with this theory, which was not yet widely accepted (Morton 1981 ), and suggested that light might be involved, which helped to pave the way for the discovery of photosynthesis. Black ( 1756 ) was able to identify carbon dioxide (which he called fixed air) using a lime water precipitation test. He demonstrated that this “fixed air” did not support animal life or a candle flame (Egerton 2008 ). Charles Bonnet ( 1754 ) made an important observation, i.e. branches with leaves that were submerged under water would produce air bubbles on their surfaces when sunlight shone on them, but not after sunset. Senebier refined these experiments in 1781 (Morton 1981 ), by showing that the leaves produced no oxygen in the sunlight when the surrounding water was free of carbon dioxide and that the rate of oxygen production was higher with carbon dioxide-saturated water. Tomic et al. ( 2005 ) present nicely the steps leading up to the term photosynthesis. This began with Priestley ( 1775 ) demonstrating that the air given off by animals and by plants was not the same, Ingen-Housz ( 1779 ) observed the important role of light, and the dispute between Senebier and Ingen-Housz from 1783 to 1789 resolved more clearly the functions of carbon dioxide emission (respiration) and absorption (photosynthesis). Based on these results and his own very detailed observations, de Saussure reported in 1804 that the carbon necessary for plant growth is absorbed mainly by green leaves from atmospheric carbon dioxide and he estimated that the largest part of the accumulated dry matter of plants is made of this carbon. Thus, the dispute of what the plant matter is made of that began in antique Greece was resolved at the end of the eighteenth century.
3 How much water do plants need to grow?
The late eighteenth century marked the beginning of applied agricultural science and the rise of plant physiology (Morton 1981 ). Work continued on transpiration and stomata, with a large number of experiments. Burgerstein ( 1887 , 1889 ) managed to assemble 236 publications on transpiration of plants from 1672 to 1886, citing short abstracts of each and comparing them critically. Also, Unger published in 1862 a major review article covering such subjects as the relationship of transpiration to temperature and humidity; daily cycles, including night; differences in adaxial and abaxial leaf surfaces; the impact on transpiration of type, number, size and distribution of stomata; the structure of the epidermis (cell layers, cuticle, hairs and wax); development of the mesophyll; size of intercellular spaces and cell turgor; and the impact of plant transpiration on the atmosphere (Unger 1862 in Burgerstein 1887 ). Scientists started to reflect on the interaction of plants, or more specifically their leaves, with their environment, and experimentation included the responses of stomata to light quantity (Möldenhawer 1812 ) and quality (Daubeny 1836 in Burgerstein 1887 ). Based on inconsistent observations by e.g. Banks, Möldenhawer and Amici, advances were also made on the functioning of stomata (Mohl 1856 ). However, progress was mainly based on a comment in von Schleiden ( 1849 ) that the state of the stomata would be the result of the water in- or outflow of the pore cells (called “Schliesszellen”) and he showed experimentally that stomata close when the pore cells lose water. As knowledge of transpiration, stomatal opening and their dependence on environmental variables increased, new questions arose about the water consumption of plants.
Another milestone along the way to understanding the transpiration of plants in the nineteenth century was the publication by Sir John Bennet Lawes ( 1850 ), “Experimental investigation into the amount of water given off by plants during their growth; especially in relation to the fixation and source of their various constituents”. He described experiments on wheat, barley, beans, peas and clover using differently fertilised soils. He was using plants in closed containers and an especially designed balance to “estimate the amounts of water given off” (Fig. 1 ). He observed increased evapotranspiration with higher temperatures during the growing season, and asked whether “this increased passage of water through the plants, carrying with it in its course many important materials of growth from the soil, and probably also influencing the changes in the leaves of these, as well as of those derived from the atmosphere, will not be accompanied with an equivalently increased growth and development of the substance of the plant”. This was followed by an important discussion of the influence of temperature on evaporation and growth as well as the resultant ratio. He discussed in the introduction “the relationship of the water given off to the matter fixed in the plants”; he gave his results in this ratio and in the inverse ratio, and applied these ratios to different scientific questions. The first ratio (transpired water divided by plant matter, the inverse of today’s TE) was used to interpret his results in terms of water use compared to field available water, and the latter’s ratio (plant matter divided by transpired water, equivalent to today’s TE) was used to discuss his results in terms of functional differences among species. From the observed functional differences, he concluded that there was “some definite relationship between the passage of water through the plants and the fixation in it of some of its constituents”. He was, thereby, introducing a new question about the link between dry matter accumulation and transpiration, which will be treated in the next chapter.
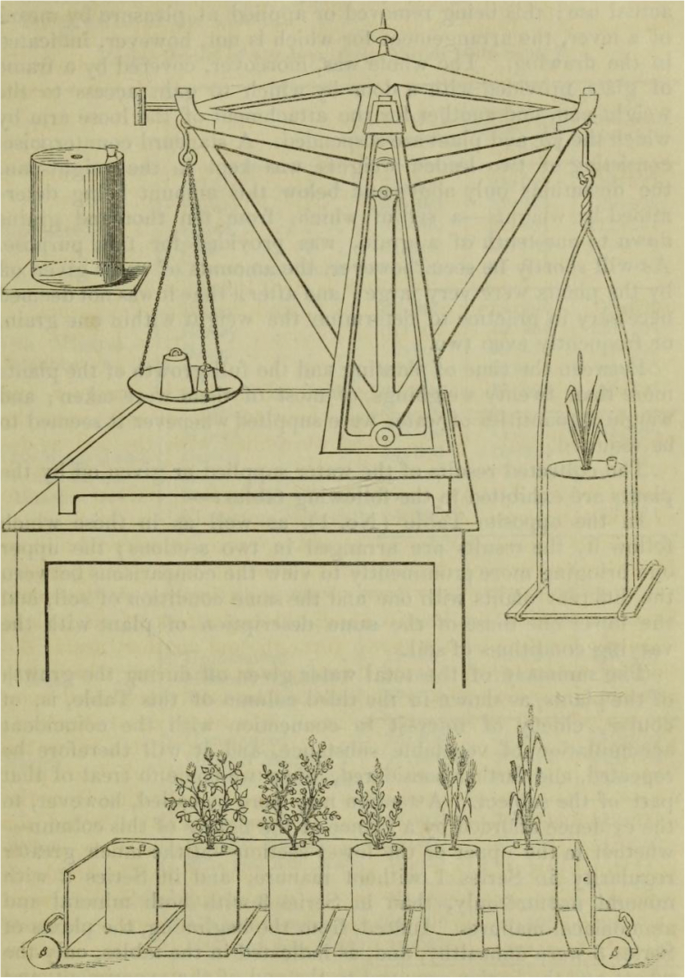
Illustration from Lawes ( 1850 , p. 43) of the special balance constructed for weighing plants in their “jars” to estimate the amounts of water given off and also the “truck” on which a series of jars was moved to the balance
Towards the end of the nineteenth century, research interest started to include agricultural questions of water use. Marié-Davy ( 1869 ) measured transpiration (standardised by leaf surface) of over 30 plant species, including eight tree or shrub species as well as herbaceous and agricultural plants. He estimated transpiration per soil area, thereby establishing that a prairie would transpire more than trees. von Höhnel ( 1879 ) estimated long-term transpiration of branches of 15 tree species (standardised on leaf surface or leaf dry weight). He used these data of branch transpiration to upscale to whole trees and concluded that compared to agricultural plants, the amount of rain seemed sufficient for tree growth. Hellriegel ( 1871 ) had already similarly concluded for cereals in the Mark Brandenburg (Germany) region that rainfall would not be sufficient, as had Marié-Davy ( 1874 ) for wheat in the Paris (France) region. In parallel with these more quantitative interrogations about water use, from the mid-nineteenth century, scientists started to ask more functional questions about the relationship between transpiration and dry matter accumulation, in a context of vigorous growth of botanical sciences and the complex relation between organisms and their environment (Morton 1981 ).
4 Are transpiration and dry matter accumulation linked?
Lawes ( 1850 ) had already reflected on a functional relationship between water flux and plant matter accumulation. In the following years, there were several publications on the transpiration of trees, and although no transpiration efficiency was estimated, the understanding of tree transpiration advanced. Many comparative studies were published. Lawes ( 1851 ) on “Comparative evaporating properties of evergreen and deciduous trees” considered twelve different tree species. He provided measurements of the variation in transpiration with temperature and hygrometry data. With these, he concluded that “evaporation is not a mere index of temperature but that it depends on vitality influenced by heat, light and other causes”. In the late nineteenth century, several researchers estimated and compared values of the ratio of transpiration and dry matter accumulation for different plants (Burgerstein 1887 ). With the growing evidence of variation in this ratio, scientists started to reflect on the relationship between transpiration and dry matter accumulation, aided by the development of new measurement techniques. A major question was if there would be a tight coupling between transpiration and dry matter accumulation, resulting in a constant transpiration efficiency, or if variation could be observed.
Dehérain ( 1869 ) studied evaporation and the decomposition of carbonic acid in leaves of wheat and barley. Using an ingenious apparatus, he was probably the first to directly measure evaporation of water in parallel with carbonic acid decomposition. He studied the effect of variously coloured light, and although he did not calculate the ratio between evaporation and carbonic acid decomposition, he did conclude that light of different colours had a similar effect on carbonic acid decomposition and on water evaporation from the leaves. His final conclusion was that “it is likely that there is existing between the two main functions of plants, evaporation and carbonic acid decomposition, a link, of which we need to determine its nature” (translation from the original French by the author). Several other scientists also commented on the relationship between transpiration and dry matter production. Fittbogen ( 1871 ) supposed, similarly to Lawes ( 1850 ) before him, but with more experimental evidence, that there should be a positive relationship between transpiration and production of dry matter. Dietrich ( 1872 in Burgerstein 1887 ) supposed that this relationship would be linear, whereas Tschaplowitz ( 1878 in Burgerstein 1887 ) introduced the idea that there should be an optimum transpiration at the maximum production of matter. Therefore, when the transpiration would increase over this optimum, this would lead to a decrease in assimilation rate. He was one of the first to suggest a non-linear relationship between transpiration and assimilation. Sorauer in “Studies on evaporation” ( 1880 ) defended the hypothesis that transpiration was not only a physical phenomenon but was also physiological. He stated that “It is not possible as yet to study the plant internal processes which regulate the transpiration, however it is possible to quantify the relationship between dry-matter and transpiration” (translation from German by the author), suggesting thereby TE as a means to advance the understanding of plant internal processes. Sorauer was probably at the cutting edge of science of his time. He pointed out specifically that variability among plants of one species was due to genetics (German, “erbliche Anlagen”), a startling and even daring assertion for his time. He asserted that for comparative studies, genetic variability needed to be minimised. To achieve this, he used, when possible, seeds from the same mother plant, grown in the same environmental conditions and a large number of repetitions. Using these protocols, he was probably one of the first to estimate TE on tree seedlings, showing that there was within species diversity in transpiration and growth, but that their ratio was more constant. He concluded from experiments on pear and apple trees that the pear trees used less water for the same biomass growth. He was able to go one step further and demonstrate that this difference was due to less transpiration per leaf area. By comparing different woody and herbaceous plants with different growth types, he postulated that when plants had a small leaf area combined with high transpiration, they had either a very strong growth increment, a high dry matter percentage, or a large root system. Overall, he observed relationships between dry matter production and transpiration; he concluded that there must be some regulation of the transpiration per unit leaf area by the co-occurring dry matter production.
Hellriegel ( 1883 ) argued that one cannot estimate a constant ratio between transpiration and production as there were factors which influence each independently. He also commented that it might make sense to estimate mean values of transpiration for various agricultural plants, as this would be for practical and scientific value. He thought that the most logical standardisation would be by the mass of the dry matter produced during the same time period. He called this “relative Verdunstungsgrösse” which can be translated into English as “relative transpiration”. He was probably one of the first to give a name to the ratio between whole plant transpiration and dry matter production. He proposed a theory that for a long-term drought, plants would acclimate their morphology to decrease their “relative transpiration”. He provided additional experimental evidence that barley had decreased in relative transpiration over as many as seven levels of soil water deficit, relative to field capacity. Using his own observations, he proposed that when calculating a mean “relative transpiration” for a single species, variation of transpiration should be minimised and that plants should be tested together only under optimal conditions. Given the relatively small differences in relative transpiration that he observed among different crops, Hellriegel suggested that these differences would not explain why some crops grow better in wet locations and others on dry locations. Hellriegel was thus probably one of the first scientists to point out that the relationship between drought adaptation and “relative transpiration” might not be straightforward.
Understanding how biomass and water loss were connected was studied by Iljin ( 1916 ) on a newly detailed level. He measured simultaneously water loss and carbon dioxide decomposition and reported his data as grammes of water lost per cubic centimetre of carbon dioxide decomposed. He concluded from studying more than 20 plant species that “...it is generally agreed that the rates of water loss and of CO 2 assimilation are directly proportionate to stomatal aperture, and that consequently there exists a close connection between these two processes”.
At the end of the nineteenth century, the ratio of transpiration versus dry matter accumulation was recognised as an important plant trait, which varies among and within species in a complex interaction of each component with the other and with environmental factors.
5 How do plants differ in water requirement and how do they respond to variations in environmental factors?
In the late nineteenth century, several researchers estimated and compared values of the ratio of transpiration and dry matter accumulation for a range of cultivated plants (Fittbogen 1871 ; Dietrich 1872 ; Farsky 1877 , cited in Burgerstein 1887 ), giving evidence of the growing interest of agricultural scientists. The number of studies of transpiration efficiency greatly increased, thereby driving a new standardisation in terminology. King ( 1889 ) studied the inverse of transpiration efficiency and described it as “the amount of water required for a ton of dry matter”, and promulgated this terminology by using it in the titles of his publications between 1892 and 1895. Similarly, Leather ( 1910 ) published “Water requirements of the crops of India”, in which he defined the “transpiration ratio” as “the water transpired to the weight of dry plant produced”. The shift from a purely descriptive use of “water requirement” to a clearly defined one was provided by Kearney and Shantz ( 1911 ) as “… the degree to which a plant is economical in its use of water is expressed in its water requirement, or the total quantity of water which it expends in producing a pound of dry matter”. The term “water requirement” is the inverse of the modern transpiration efficiency, and was used by a rapidly increasing number of publications which were published on the water use of crops in the early twentieth century. Montgomery ( 1911 ) may have been the first to use the term for a plant trait in “Methods of determining the water requirements of crops”.
At the beginning of the twentieth century, the importance of gaining knowledge on the water requirements of plants can be seen in the technical effort that went into the measuring equipment. von Seelhorst ( 1902 ) presented a system of growing boxes on rails, placed belowground, including the balance, so that the top of the growing boxes was at the same level as the surrounding soil (Fig. 2 ). In the now well-known studies on “The water requirement of plants. I. Investigations in the Great Plains in 1910 and 1911”, Briggs and Shantz ( 1913a ) measured the water requirement for 21 crop and weed species, sometimes for different varieties of the same crop and under controlled and field conditions. In the same year, they reviewed the available literature on water requirement (Briggs and Shantz 1913b ), increasing their dataset to 31 different crop species. They discussed in detail studies from 29 different authors, many of which had only published once or twice on this subject. A few researchers were notable for their number of publications on the water requirement of crop plants: King with 6 publications between 1889 and 1905, and von Seelhorst with 9 publications between 1899 and 1907. The largest contributions came from Hellriegel ( 1883 ; 10 species) and Leather ( 1911 ; 15 species). Kiesselbach ( 1916 ) also reviewed 59 publications from 1850 to 1915 “which had studied transpiration in relation to crop yield, based upon plants grown beyond the seedling stage”. There were regular publications of original work from 1870s onwards, with more than one publication per year from 1890 onwards. The difference among species and the impact of environmental factors on water requirement was one of the main questions raised. These reviews and the increasing amount of newly published work per year are evidence of the growing interest in the “water requirement” of plants as a trait of increasing importance in agricultural sciences.
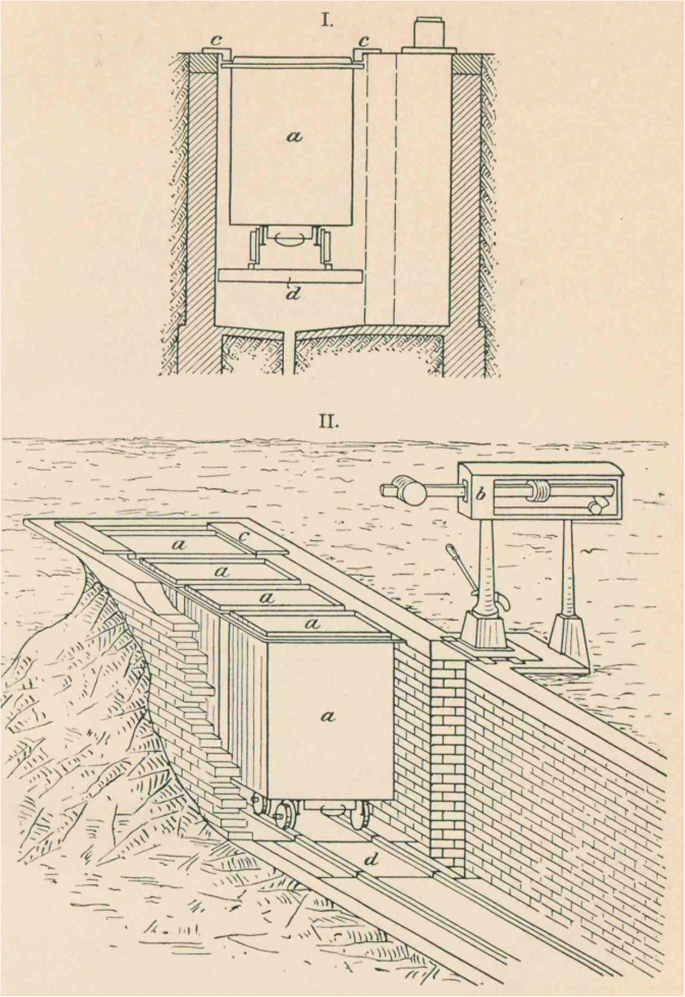
Illustration from von Seelhorst ( 1902 ), showing the quite sophisticated outdoor installation “Vegetationskasten” (growing boxes, translations by the author) to weigh plants in small waggons, with a “Kastenwagen” (boxwaggon), b “Waagebalken” (scale beam), c “Deckbretter” (cover board) and d “Waagentisch” (weighing table)
With regard to species differences in water requirement among crops, Schröder ( 1895 , cited in Maximov 1929 ) found two groups, among seven cereals, which differed in water requirement by a factor of 2. Millet, sorghum and maize were known to be drought resistant, and showed a lower water requirement than the remaining plants. These differences were confirmed by Kolkunov ( 1905 , cited in Maximov 1929 ), Briggs and Shantz ( 1914 ), Briggs and Shantz ( 1917 ) and Shantz ( 1927 ). Millet, sorghum and maize are now known to use the C4 carbon pathway of photosynthesis.
With regard to external environmental influences on plants, Briggs and Shantz ( 1913b ) distinguished between soil, atmosphere and plant factors. Soil factors which were investigated were soil moisture content, soil type, cultivation, soil volume, soil temperature, effect of fertilisers in soil or water cultures and effect of previous crops. Weather factors considered were air temperature and humidity, shade and carbon dioxide content. Other factors studied in direct relationship to the plants were parasite attacks, relative leaf area, cutting frequency, defoliation, planting density and the age of plants.
A critique of the term “water requirement” was not long in coming. Dachnowski ( 1914 ) wrote, “It is assumed by many writers that a definite and quantitative relation exists between transpiration and growth, and that hence the ratio of the weight of water absorbed and transpired by a plant during its growth to the green or dry substance produced is an adequate and simple measure of growth.”, followed by an argument why this was not the case.
6 Why do plants differ in transpiration efficiency?
The adaptations of plants to dry environments were an important ecological topic at the beginning of the twentieth century, as the discipline of “physiological ecology” (Iljin 1916 ; Moore 1924 ) began to develop. Iljin ( 1916 ) studied more than 20 different plant species in situ from different ecological locations, e.g. wet bottom soils and variously facing slopes of ravines with different aspects. Iljin proposed that “the water requirements of the different species should be very different, and consequently the amounts of water available should differently affect their processes of life”. Using his observations, he was able to show that “… in no case was the water loss per unit of decomposed CO 2 found to be equal to or more in xerophytes than in mesophytes”, thus suggesting a higher transpiration efficiency. He argued that mesophytes would have to close stomata “… in dry places in order to reduce evaporation, thus diminishing the rate of assimilation as well, whereas in the case of xerophytes, which are adapted to extreme conditions of existence, assimilation in similar circumstances proceeds actively”. He then tried to confirm his hypothesis by transplanting mesophytes from wetter sites to the drier environment of xerophytes. Iljin showed experimentally that in all cases, a higher water requirement was measured for mesophytes transferred to a drier site compared to their original site and compared to xerophytes at the dry site. He interpreted his observations as “plants growing in dry places are adapted to a more economical consumption of water”. He held this to be true for among- and within-species variation.
A milestone in forest “physiological ecology” was Bates’ ( 1923 ) study of the physiological requirements of Rocky Mountain trees. Bates wrote that for foresters, knowledge of demands of tree seedlings for moisture, light, heat and soil fertility was important for planning reforestation. He started a large investigation of six forest tree species, combining field studies to describe ecosystems, with experiments in controlled environments in order to determine species differences in relative transpiration and other water flow-related traits. Bates concluded from the comparison among species that trees of low water requirement would be trees that have a superior control over their water supply. He was however critical of a direct relationship between water requirement and drought resistance in trees. Moore ( 1924 ) commented that in correlating physiological measurements with the habitat characterisation of the species, Bates “... has opened new fields to forest investigations”. He also stressed that the results were counterintuitive in that the most xerophytic species had the highest water requirement, whereas the most mesophytic species had the lowest water requirement.
A similar discrepancy was observed by Maximov ( 1929 ) in the chapter “Efficiency of transpiration” in his book The Plant in relation to water , which was translated from Russian into English rapidly after its publication. Maximov preferred “efficiency of transpiration” to “water requirement”, arguing that the former would be more logically correct, because the determining process (transpiration) should be in the denominator, which also would have the effect that “… an increase in the figure denoting the value of the ratio actually corresponds to an increase of the efficiency per unit of water used”.
In his book, Maximov ( 1929 ) described experiments done at Tiflis Botanic garden (today in Georgia) by Maximov and Alexandrov ( 1917 ), where they studied local xerophytes for 3 years. They found xerophytes with a high efficiency of transpiration, particularly drought-resistant annuals. They also found that plants with a low efficiency of transpiration appeared to be the most typical semi-arid xerophytes. The mesophytes all displayed a medium efficiency. Maximov noted from other observations on the same plants that the “… majority of xerophytes with a low efficiency of water expenditure possess very extensive root systems, far exceeding in length the sub-aerial portions of the plant”. He also observed that these plants showed a strong transpiration and that this transpiration might constitute the “pump” which could draw water through such an extensive root system. He also observed that “members of the group of annual xerophytes with a high efficiency of transpiration are characterised by a relatively large leaf surface, which develops very rapidly”. He argued that this would confer a high intensity of assimilation. From these observations, he concluded a “lack of direct proportionality between efficiency of transpiration and the degree of drought resistance”, but also that “the magnitude of the efficiency of transpiration affords one of the most satisfactory tests of the ecological status of a plant”. Maximov applied the ecological classification developed by Kearney and Shantz ( 1911 ), which they had based on plants of the arid and semi-arid regions of North America: (1) drought-escaping with an annual growth cycle restricted to favourable conditions; (2) drought-evading, delay by various means the exhaustion of soil moisture; (3) drought-enduring, can wilt or dry but remains alive; and (4) drought-resisting, can store a water supply. It should be noted that the ecological definitions behind these concepts have changed with time and are used slightly differently today. Shantz ( 1927 ) argued that many of the drought-evading plants had a low water requirement and Maximov noted that this group included the highly efficient xerophytes with a large leaf area. Maximov also observed that xerophytes from the third group (drought-enduring) could show a very low efficiency of transpiration and belonged to the group of xerophytes with large root systems. Without concluding directly, he suggested a relationship between the transpiration efficiency of a xerophyte and its ecological strategy when facing limited soil water content. These studies by Maximov are among the most complete concerning the relationship between a plants’ resistance to drought and their transpiration efficiency, reflecting the interest of scientists in ecological questions of plant functioning, especially in relation to drought.
Although work on crop plants advanced greatly in the early twentieth century, results were scarcer for tree species. Raber ( 1937 ) concluded his book on “Water utilization by trees, with special reference to the economic forest species of the north temperate zone” with detailed discussions of available data for forest trees. He commented that “much more work on the water requirements of trees of all ages and under varying site conditions is needed”. And he continued that “In view of the importance of planting drought-resistant species in regions where the water supply is below the optimum for most tree species, it is extremely urgent to know more about what qualities make for drought resistance and what species possess these qualities to the greater degree.” These conclusions by Raber show that from the beginning of the twentieth century, the estimation of transpiration efficiency had taken an important place in ecological studies on forest tree species, however not without some critical thoughts on the subject.
7 What is the functional importance of transpiration?
Already in the 1870s and 1880s, the role of stomata in the diffusion of carbon dioxide into the leaf (during the day) and out of the leaf (during the night) was discussed in the scientific literature, as shown by the extensive literature review by Blackman ( 1895 ) (see also section 4 above). Especially the functional importance of transpiration was an open question. There were two opposing lines of thought. As summarised by Iljin ( 1916 ), one defended the line of inquiry that transpiration was important only in the process of transporting mineral salts from roots to leaves; the other held that the opening of stomata was necessary for absorbing the carbonic acid from the atmosphere, which leads to a loss of water and is described as an “inevitable evil”. Iljin ( 1916 ) preferred the second line of investigation and attributed a major role to the stomatal aperture, which controlled both the absorption of carbonic acid from the atmosphere and the loss of water. He concluded that in “physiologico-ecological” investigations, assimilation should be studied together with transpiration. Maskell published a series of papers in 1928, where especially “XVIII.—The relation between stomatal opening and assimilation.” (Maskell and Blackman 1928 ) used an apparatus to estimate apparent CO 2 assimilation and transpiration rate in parallel (Fig. 3 ), and was therefore able to study in detail their interdependence, developing the first mathematical descriptions, based on the development of the theories about the diffusion of gases (Brown and Escombe 1900 ). Methodological advances intensified research on the leaf-level relationship between assimilation and transpiration and allowed the study of plant functioning in more detail. The major step forward was the construction of an infrared gas analyser (URAS: in German “Ultrarotabsorptionsschreiber”, IRGA, infrared gas analyser) by Lehrer and Luft in 1938 (Luft 1943 ) at a laboratory of BASF, IG Farbenindustrie. Normally used in industry and mining, Egle and Ernst ( 1949 ) may have been the first to describe the use of the URAS for plant physiological measurements. By 1959, the URAS was routinely used for measuring stomatal resistance or transpiration in parallel and simultaneously with CO 2 assimilation, on the same leaf (Rüsch 1959 ). This was a great improvement on previous methods and led rapidly to a set of equations for calculating assimilation and stomatal conductance (Gaastra 1959 ).
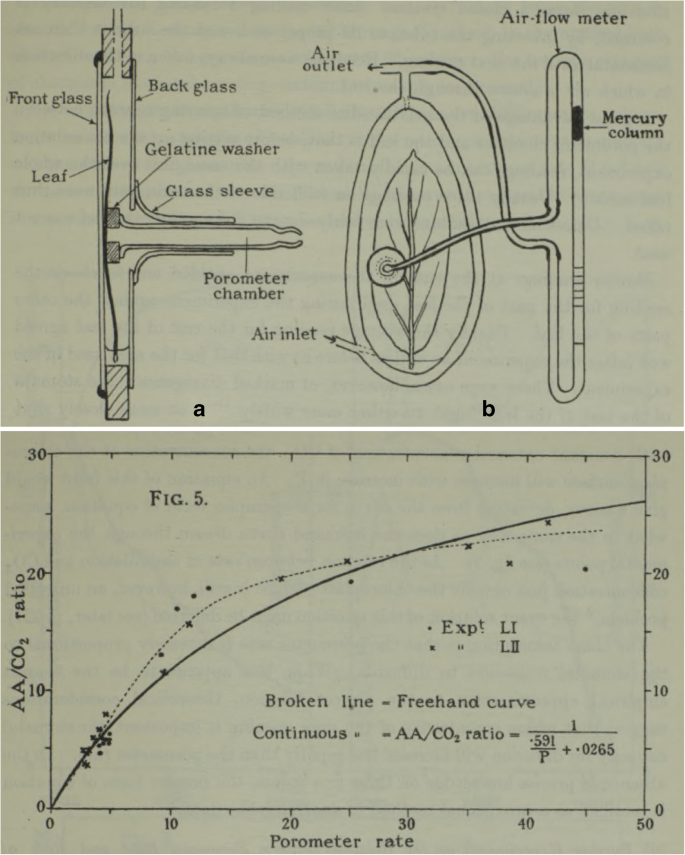
Two figures taken from Maskell and Blackman ( 1928 ): on the top, Figure 1 (p. 489) showing a “Combined assimilation chamber and porometer for simultaneous investigation of apparent assimilation and stomatal behaviour. A. Section of leaf chamber passing through porometer chamber. B. Back view of leaf chamber showing also air-flow meter attached by pressure tubing to porometer and to leaf chamber”. On the bottom, Figure 5 (p. 497) “Relation between porometer rate and apparent assimilation at ‘high’ light, December 1920.” Exp t LI and LII correspond to 2 days of continuous measurements to what Maskell called “diurnal march”
Scarth ( 1927 ) argued that there would be little advantage for a plant to have a high rate of transpiration, but stressed the “... advantage of maintaining the fullest diffusive capacity of the stomata and the highest possible pressure of CO 2 in the intercellular spaces”. He concluded that the principal function of stomata “... is to regulate that very factor which is presumed to regulate them, viz. the concentration of CO 2 in the leaf or, respectively, in the guard cells”. Maskell and Blackman ( 1928 ) tested this hypothesis experimentally and concluded that the rate of uptake of carbon dioxide was determined by variations in stomatal resistance and by resistances within the leaf, thereby introducing the importance of the CO 2 concentrations in the chloroplasts. The suggestion of a strong link between the leaf internal carbon dioxide concentration and leaf-level WUE represented a large advance in the theoretical understanding of WUE.
Penman and Schofield ( 1951 ) proposed, perhaps, the first theoretical link between the leaf-level transpiration ratio (leaf transpiration divided by assimilation) and the ratio of the coefficients of diffusion of water vapour and carbon dioxide in air, and the water vapour and carbon dioxide air-to-leaf pressure gradients. Gaastra ( 1959 ) suggested that the leaf internal conductance to carbon dioxide is a pivotal point of the ratio of assimilation to transpiration and of the water economy of crop plants. Bierhuizen and Slatyer ( 1965 ) showed that the transpiration ratio could be predicted from water vapour and carbon dioxide gradients over a range of light intensities, temperatures and relative humidities. These studies were the first to suggest that whole plant transpiration efficiency might be regulated directly by leaf functioning and would be therefore a trait in its own right and not only the ratio of two plant traits.
8 How can the transpiration ratio be improved?
Because water is increasingly scarce in a warming world, Rüsch ( 1959 ) queried whether the luxury of highly transpiring tree species could be justified. He argued for selective breeding of tree species varieties with low transpiration-to-assimilation ratio T/A by means of minimising transpiration while maximising assimilation. Also Polster et al. ( 1960 ) assessed the potential suitability of tree species to sites by their dry matter production and transpiration ratio. Troughton ( 1969 ) and Cowan and Troughton ( 1971 ) suggested that genetic selection of plant varieties could be used to improve the transpiration ratio by decreasing leaf internal resistance to carbon dioxide diffusion. Cowan and Farquhar ( 1977 ) built on this theme by proposing that stomata might optimise carbon gain to water lost by varying the conductances to diffusion and thereby maximising the ratio of the mean assimilation rate to mean rate of evaporation in a fluctuating environment. Approaches which target photosynthesis, stomatal opening, leaf internal resistance to carbon dioxide diffusion or stomatal optimisation in order to improve plants performance have since been followed in plant breeding and have largely been reviewed elsewhere (e.g. Condon et al. 2004 ; Cregg 2004 ; Vadez et al. 2014 ).
9 Intrinsic water use efficiency and carbon stable isotopes
Another milestone towards contemporary research on water use efficiency was the use of stomatal conductance to water vapour rather than transpiration by Farquhar and Rashke ( 1978 ) and to calculate water use efficiency as assimilation divided by stomatal conductance. This definition allowed an estimation of water use efficiency resulting only from plant functioning, without a direct impact from leaf-to-air vapour pressure difference and was named by Meinzer et al. ( 1991 ) “intrinsic water use efficiency” (W i ). Knowledge of W i facilitated the search for a genetic basis of within species variation, e.g. Brendel et al. ( 2002 ), Condon et al. ( 2002 ) and Chen et al. ( 2011 ).
Development of the stable carbon isotope method for estimating W i resulted in a widely applicable screening method, and a large increase of publications around plant water use efficiency. Based on the two-step fractionation model (atmospheric CO 2 – leaf internal CO 2 – plant carbon) proposed by Park and Epstein ( 1960 ), various models explaining the difference in carbon isotope composition between atmospheric CO 2 and plant carbon were developed in the late 1970s and early 1980s, e.g. Grinsted ( 1977 ), Schmidt and Winkler ( 1979 ) and Vogel ( 1980 ). Vogel’s model contained many theoretical aspects which, however, lacked experimental understanding. In parallel, Farquhar ( 1980 ) developed a similar model, but which resulted in a simple, elegant mathematical equation relating plant natural abundance carbon isotope discrimination, relative to atmosphere, to the ratio of leaf internal to atmospheric CO 2 concentration. This was, in turn, related to W i . Experimental evidence showed that carbon isotope measurements, in wheat, reflected long-term water use efficiency (Farquhar et al. 1982 ) as well as whole plant transpiration efficiency (Farquhar and Richards 1984 ). They concluded that carbon isotope discrimination may provide an effective means to assess and improve WUE of water-limited crops. Strong correlations between whole plant TE and stable carbon isotope measurements of plant organic material were shown in a host of papers to be. Some of these papers were (1) for crops and other annuals (Hubick et al. 1986 ; Ehleringer et al. 1990 ; Virgona et al. 1990 ) and (2) for trees (Zhang and Marshall 1994 ; Picon et al. 1996 ; Roupsard et al. 1998 ). The isotopic method has spread rapidly as a general estimator of WUE and continues to be used widely in screening programmes for plant improvement as well as in ecological research, e.g. Rundel et al. ( 1989 ) and notably used in tree rings (McCarroll and Loader 2004 ).
10 Closing remarks
Water use efficiency is probably one of the oldest of plant traits to stimulate across the centuries the interest of philosophers, theologians, Middle Age savants, natural philosophers and modern plant scientists across different disciplines (plant physiology, ecophysiology, ecology, genetics, agronomy). The interest began as a purely philosophical one, progressed to thought experiments, towards an interest in plant functioning and its relationship to the environment.
Already in the early Renaissance (mid-fifteenth century), an experimentation was proposed, in a time when botany consisted mainly of naming plants (Morton 1981 ). It is then also an early example of an actually performed experimentation, the famous willow experiment by Van Helmont ( 1662 ) as well as of early “in laboratory” experimentation on plants (hydroponics experiments by Woodward 1699 ). The question of what makes plants grow, between water and soil, kept natural philosophers busy up to the end of the eighteenth century, when the assimilation of CO 2 was discovered and the question finally solved.
Early in the nineteenth century, the interest and experimentation turned to the amount of water that plants would need to grow, in the context of a developing research on agricultural practices (Morton 1981 ). Biomass was used to standardise the water losses which allowed comparisons among species (crops as well as trees) and a beginning study of the impact of different environmental variables.
At the end of the nineteenth century, knowledge on the physiological aspects of CO 2 assimilation and the control of transpiration by stomata had sufficiently advanced, so that scientists started to reflect on their inter-dependency. Was transpiration only a physical process or was there a physiological control? Was transpiration regulated by the dry matter production? Or does the stomatal opening determine the rate of CO 2 assimilation?
At the turn of the twentieth century, the study of species differences led to questioning why these differences did exist. As the discipline of “physiological ecology” developed, “water requirement” was inverted into an “efficiency”, reflecting an evolution from standardising transpiration to a trait in its own right. This introduced ecological questions about the adaptation of plants to dry environments and the relation to transpiration efficiency. Counterintuitive results stimulated the discussion and linked differences in WUE to different ecological strategies.
Methodological and theoretical advances in the description of leaf gas exchange in the mid-twentieth century showed the central role of stomata in the control of transpiration and CO 2 assimilation, leading to the idea that stomata might optimise water losses versus carbon gain. The development of carbon stable isotopes as an estimator of leaf-level WUE was an important step not only to further develop these theoretical considerations, but also towards large-scale studies. In parallel, modelling approaches were developed to scale from leaf-level WUE to whole plant TE, e.g. Cernusak et al. ( 2007 ), and to the field or canopy, e.g. Tanner and Sinclair ( 1983 ).
At least from the beginning of the twentieth century onwards, also critical views on the relationship between water requirement and its relation to growth mostly in terms of yield were published (Dachnowski 1914 ). Viets ( 1962 ) asked “Is maximum water use efficiency desirable?”, especially in terms of crop production. Sinclair et al. ( 1984 ) considered different options for improving water use efficiency, however concluding that most of these have important limitations or drawbacks. This discussion is ongoing, as can be seen by the article published by Blum ( 2009 ): “Effective use of water (EUW) and not water-use efficiency (WUE) is the target of crop yield improvement under drought stress”.
Exploration and application of transpiration efficiency at the whole plant level, and its derivatives at other levels, are still a very active research field across nearly all levels of forest science: concerning very rapid processes at the leaf level (Vialet-Chabrand et al. 2016 ), up-to-date genetic and genomic approaches for breeding (Plomion et al. 2016 ; De La Torre et al. 2019 ; Vivas et al. 2019 ), studying local adaptation of trees to their environment in a population genetic context (Eckert et al. 2015 ) or an ecological context (Pellizzari et al. 2016 ), water use efficiency from the plant to the ecosystem (Medlyn et al. 2017 ), estimated at the population level (Rötzer et al. 2013 ; Dekker et al. 2016 ) or modelling up to the global earth level (Cernusak et al. 2019 ), just to name a few. Thus, the first curiosity of Greek philosophers has motivated scientists through history, with many exciting discoveries still to come.
Change history
17 june 2021.
A Correction to this paper has been published: https://doi.org/10.1007/s13595-021-01073-0
Bates CG (1923) Physiological requirements of Rocky Mountain trees. J Agric Res 24:97–164
Google Scholar
Bierhuizen J, Slatyer R (1965) Effect of atmospheric concentration of water vapour and CO 2 in determining transpiration-photosynthesis relationships of cotton leaves. Agric Meteorol 2:259–270
Article Google Scholar
Black J (1756) Experiments upon magnesia alba, quicklime, and some other alkaline substances. Essays Obs Phys Lit 2:157–225
Blackman F (1895) XI. Experimental researches on vegetable assimilation and respiration.—No. II. On the paths of gaseous exchange between aerial leaves and the atmosphere. Philos Trans R Soc B 186:503–562
Blum A (2009) Effective use of water (EUW) and not water-use efficiency (WUE) is the target of crop yield improvement under drought stress. F Crop Res 112:119–123
Bonnet C (1754) Recherches sur l’Usage des feuilles dans les plantes. Elie Luzac, Fils, Göttingen
Boyle R (1661) The sceptical chymist. J. Cadweill for J. Crooke
Brendel O, Pot D, Plomion C, Rozenberg P, Guehl JM (2002) Genetic parameters and QTL analysis of ẟ 13 C and ring width in maritime pine. Plant Cell Environ 25:945–953
Article CAS Google Scholar
Briggs LJ, Shantz HL (1913a) The water requirement of plants. I. Investigations in the Great Plains in 1910 and 1911. US Dep Agric Bur Plant Ind Bull 284:1–48
Briggs LJ, Shantz HL (1913b) The water requirement of plants. II. A review of the literature. US Dep Agric Bur Plant Ind Bull 285:1–96
Briggs LJ, Shantz HL (1914) Relative Water Requirement of Plants. J Agric Res 3:1–64
CAS Google Scholar
Briggs LJ, Shantz HL (1917) The water requirement of plants as influenced by environment. In: Proceedings of the Second Pan American Scientific Congress. Pp 95–107
Brown HR (2013) The theory of the rise of sap in trees: some historical and conceptual remarks. Phys Perspect 15:320–358
Brown H, Escombe F (1900) VIII. Static Diffusion of gases and liquids in relation to the assimilation of carbon and translocation in plants. Philos Trans R Soc B 193:223–291
Brown P, Shrader W (1959) Grain yields, evapotranspiration, and water use efficiency of grain sorghum under different cultural practices. Agron J 51:339–343
Bugler G (1950) Un précurseur de la biologie expérimentale: Edme Mariotte. Rev Hist Sci (Paris) 3:242–250
Burgerstein A (1887) Materialien zu einer Monographie betreffend die Erscheinungen der Transpiration der Pflanzen. Verhandlungen der Zool Gesellschaft Wien 37:691–782
Burgerstein A (1889) Materialien zu einer Monographie, betreffend die Erscheinungen der Transpiration der Pflanzen. II. Theil. Verhandlungen der Zool Gesellschaft Wien 39:399–464
Cernusak LA, Aranda J, Marshall JD, Winter K (2007) Large variation in whole-plant water-use efficiency among tropical tree species. New Phytol 173:294–305
Article PubMed Google Scholar
Cernusak LA, Haverd V, Brendel O et al (2019) Robust response of terrestrial plants to rising CO 2 . Trends Plant Sci 24(7):578–586 1–9
Article CAS PubMed Google Scholar
Chen J, Chang SX, Anyia AO (2011) Gene discovery in cereals through quantitative trait loci and expression analysis in water-use efficiency measured by carbon isotope discrimination. Plant Cell Environ 34:2009–2023
Condon AG, Richards RA, Rebetzke GJ, Farquhar GD (2002) Improving intrinsic water-use efficiency and crop yield. Crop Sci 42:122–131
PubMed Google Scholar
Condon AG, Richards RA, Rebetzke GJ, Farquhar GD (2004) Breeding for high water-use efficiency. J Exp Bot 55:2447–2460
Cowan IR, Farquhar GD (1977) Stomatal function in relation to leaf metabolism and environment. In: Integration of activity in the higher plant. University Press, pp 471–505
Cowan IR, Troughton J (1971) The relative role of stomata in transpiration and assimilation. Planta 97:325–336
Cregg B (2004) Improving drought tolerance of trees: theoretical and practical considerations. In: Acta Horticulturae Evaluation, Production and Use, XXVI International Horticultural Congress: Nursery Crops; Development. Aug 11-17, 2002, pp 147–158
Dachnowski A (1914) Transpiration in relation to growth and to the successional and geographical distribution of plants. Ohio Nat 14:241–251
Daubeny C (1836) On the action of light upon the atmosphere. Philos Trans R Soc 126:149–175
De La Torre A, Puiu D, Langley CH et al (2019) Genomic architecture of complex traits in loblolly pine. New Phytol 221:1789–1801
de Saussure N (1804) Chemische Untersuchungen über die Vegetation. Leipzig, 1890
Dehérain M (1869) L’évaporation de l’eau et la decomposition de l’acide carbonique par les feuilles des végétaux. Aannales des Sci Nat–Bot 5(XVII):5–23
Dekker SC, Groenendijk M, Booth BBB, Huntingford C, Cox PM (2016) Spatial and temporal variations in plant water-use efficiency inferred from tree-ring, eddy covariance and atmospheric observations. Earth Syst Dyn 7:525–533
Dietrich T (1872) Ueber die durch unsere Culturpflanzen verdunsteten Wassermengen. Mitth des landw Cent für den Regierungsbezirk Cassel 1872:343
Dreibelbis F, Harrold L (1958) Water-use efficiency of corn, wheat, and meadow crops. Agron J 50:500–5003
Eckert AJ, Maloney PE, Vogler DR, Jensen CE, Mix AD, Neale DB (2015) Local adaptation at fine spatial scales: an example from sugar pine ( Pinus lambertiana , Pinaceae ). Tree Genet Genomes 11:42
Egerton FN (2001a) A history of the ecological sciences: early Greek origins. Bull Ecol Soc Am 82:93–97
Egerton FN (2001b) A history of the ecological sciences, part 4: Roman natural history. Bull Ecol Soc Am 82:243–246
Egerton FN (2002) A history of the ecological sciences, part 7: Arabic language science: botany, geography, and decline. Bull Ecol Soc Am 83:261–266
Egerton FN (2003) A history of the ecological sciences, part 10: botany during the Italian Renaissance and beginnings of the scientific revolution. Bull Ecol Soc Am 84:130–137
Egerton FN (2004a) A history of the ecological sciences, part 12: invertebrate zoology and parasitology during the 1500s. Bull Ecol Soc Am 85:27–31
Egerton FN (2004b) A history of the ecological sciences, part 13: broadening science in Italy and England, 1600–1650. Bull Ecol Soc Am 85:110–119
Egerton FN (2004c) A history of the ecological sciences, part 14: plant growth studies in the 1600s. Bull Ecol Soc Am 85:208–213
Egerton FN (2005) A history of the ecological sciences, part 16: Robert Hooke and the Royal Society of London. Bull Ecol Soc Am 86:93–101
Egerton FN (2008) A history of the ecological sciences, part 28: plant growth studies during the 1700s. Bull Ecol Soc Am 89:159–175
Egle K, Ernst A (1949) Die Verwendung des Ultrarotabsorptionsschreibers für die vollautomatische und fortlaufende CO 2 -Analyse bei Assimilations-und Atmungsmessungen an Pflanzen. Zeitschrift für Naturforsch B 4:351–360
Ehleringer J, White J, Johnson D, Brick M (1990) Carbon isotope discrimination, photosynthetic gas exchange, and transpiration efficiency in beans and range grasses. Acta Oecol 11:611–625
Farquhar G (1980) Carbon isotope discrimination by plants: effects of carbon dioxide concentration and temperature via the ratio of intercellular and atmospheric CO 2 concentrations. In: Carbon dioxide and climate: Australian research. Australian Academy of Science, Canberra, pp 105–110
Farquhar GD, Rashke K (1978) On the resistance to transpiration of the sites of evaporation within the leaf. Plant Physiol 61:1000–1005
Article CAS PubMed PubMed Central Google Scholar
Farquhar GD, Richards PA (1984) Isotopic composition of plant carbon correlates with water-use efficiency of wheat genotypes. Aust J Plant Physiol 11:539–552
Farquhar GD, O’Leary MH, Berry JA (1982) On the relationship between carbon isotope discrimination and the intercellular CO2-concentration in leaves. Aust J Plant Physiol 9:121–137
Farsky F (1877) Ueber die Wasserverdunstung von Korn, Gerste und Erbse. Chem List [Chemische Blätter] tom I
Fittbogen J (1871) Altes und Neues aus dem Leben der Gerstenpflanze. Landwirtsch Versuchs-Stationen 13:81–136
Gaastra P (1959) Photosynthesis of crop plants as influenced by light, carbon dioxide, temperature, and stomatal diffusion resistance. Meded van Landbouwhoogeschool Wageningen 59:1–68
Govindjee, Krogmann D (2004) Discoveries in oxygenic photosynthesis (1727-2003): a perspective. Photosynth Res 80:15–57
Grew N (1682) The anatomy of plants. W. Rawlins, London
Grinsted M (1977) A study of the relationships between climate and stable isotope ratios in tree rings. University of Waikato PhD Thesis
Hales S (1727) Vegetable staticks, or, an account of some statical experiments on the sap in vegetables : being an essay towards a natural history of vegetation : also, a specimen of an attempt to analyse the air, by a great variety of chymio-statical experiments. W. and J Innys and T Woodward, London
Hellriegel (1871) Wie viel Wasser beanspruchen unsere Getreidearten zur Production einer vollen Ernte? Amtliches Vereinsblatt des landwirtlischaftlichen Prov fuer die Mark Brand und Niederlausitz 3:60–62
Hellriegel H (1883) Beiträge zu den Naturwiss. Grundlagen des Ackerbaus. F, Vieweg und Sohn, Braunschweig
Hershey D (2003) Misconceptions about Helmont’s willow experiment. Plant Sci Bull 49:78–83
Hobart C, Harris K (1946) Fitting cropping systems to water supplies in Central Arizona. College of Agriculture, University of Arizona, Tucson, AZ, USA
Hopkins J (1996) Nicholas of Cusa on wisdom and knowledge. Arthur Banning Press, Minneapolis
Hubick K, Farquhar G, Shorter R (1986) Correlation between water-use efficiency and carbon isotope discrimination in diverse peanut ( Arachis ) germplasm. Aust J Plant Physiol 13:803–816
Iljin V (1916) Relation of transpiration to assimilation in steppe plants. J Ecol 4:65–82
Ingen-Housz J (1779) Experiments upon vegetables, discovering their great power of purifying the common air in the sunshine and of injuring it in the shade and at night. P. Elmsly, and H. Payne, London
Kearney TH, Shantz HL (1911) The water economy of dry-land crops. Yearb United States Dep Agric 10:351–362
Kiesselbach T (1916) Transpiration as a factor in crop production. Bull Agric Exp Stn Nebraska 6:19–38
King F (1889) Soil physics. Annu Rep Agric Exp Stn Univ Wisconsin 6:189–206
Kolkunov W (1905) Contributions to the problem of breeding drought resistant crop plants. I. Anatomical and Physiological investigations of the degree of xerophily of certain cereals. Mém Polytech Inst Kiev 5(4):
Lavoisier A-L (1770) Sur la nature de l’eau et sur les expériences par lesquelles on a prétendu prouver la possibilité de son changement en terre. Mémoires l’Académie des Sci:73–82
Lawes JB (1850) Experimental investigation into the amount of water given. J Hortic Soc London 5:38–63
Lawes JB (1851) Report upon some experiments undertaken at the suggestion of Professor Lindley, to ascertain the comparative evaporating properties of evergreen and deciduous trees. J Hortic Soc London 6:227–242
Lazenby EM (1995) The historia plantarum generalis of John Ray: book i - a translation and commentary. Newcastle University PhD thesis
Leather JW (1910) Water requirements of crops in India. Mem Dep Agric India Chem Ser 1(3):133–154
Leather JW (1911) Water requirements of crops in India. -II. Mem Dep Agric India Chem Ser 1:205–281
Lévesque M, Siegwolf R, Saurer M, Eilmann B, Rigling A (2014) Increased water-use efficiency does not lead to enhanced tree growth under xeric and mesic conditions. New Phytol 203:94–109
Linares J, Camarero J (2012) From pattern to process: linking intrinsic water-use efficiency to drought-induced forest decline. Glob Chang Biol 18:1000–1015
Luft K (1943) Über eine neue Methode der registrierenden Gasanalyse mit Hilfe der Absorption ultraroter Strahlen ohne spektrale Zerlegung. Z Tech Phys 24:97–104
Malpighi M (1675) Anatome Plantarum. Johannis Martyn, London
Marié-Davy H (1869) Evaporation du sol et des plantes. J d’Agriculture Prat 2:234–239
Marié-Davy H (1874) Note sur la quantité d’eau consommée par le froment pendant sa croissance. Comptes rendus Hebd des séances l’Académie des Sci 79:208–212
Maskell EJ, Blackman FF (1928) Experimental researches on vegetable assimilation and respiration. XVIII.—The relation between stomatal opening and assimilation.—A critical study of assimilation rates and porometer rates in leaves of Cherry Laurel. Proc R Soc Lond Ser B 102:488–533
Maximov NA (1929) The plant in relation to water. George Allen & Unwin LTD, London
Maximov NA, Alexandrov V (1917) The water requirement and drought resistance of plants. Trav du Jard Bot Tiflis 19:139–194
McCarroll D, Loader NJ (2004) Stable isotopes in tree rings. Quat Sci Rev 23:771–801
Medlyn BE, De Kauwe MG, Lin YS et al (2017) How do leaf and ecosystem measures of water-use efficiency compare? New Phytol 216:758–770
Meinzer FC, Ingamells JL, Crisosto C (1991) Carbon isotope discrimination correlates with bean yield of diverse coffee seedling populations. Hort Sci 26:1413–1414
Möbius M (1901) Die Anatomie der Pflanzen I: and II. Theil. Engelmann, W, Leipzig
Mohl H (1856) Welche Ursachen bewirken die Erweiterung und Verengung der Spaltöffnungen? Bot Zeitung 14:697–704
Möldenhawer J (1812) Beyträge zur Anatomie der Pflanzen. CL Wäser, Kiel
Montgomery E (1911) Methods of determining the water requirements of crops. Proc Am Soc Agron 3:261–283
Moore B (1924) Reviewed work: Physiological requirements of Rocky Mountain trees by Carlos G. Bates Ecology 5:298–302
Morton A (1981) History of botanical science: an account of the development of botany from ancient times to the present day. Academic Press, London
Nickelsen K (2007) From leaves to molecules: botany and the development of photosynthesis research. Ann Hist Philos Biol 12:1–40
Park R, Epstein S (1960) Carbon isotope fractionation during photosynthesis. Geochim Cosmochim Acta 21:110–126
Pellizzari E, Camarero JJ, Gazol A, Sangüesa-Barreda G, Carrer M (2016) Wood anatomy and carbon-isotope discrimination support long-term hydraulic deterioration as a major cause of drought-induced dieback. Glob Chang Biol 22:2125–2137
Penman HT, Schofield RK (1951) Some physical aspects of assimilation and transpiration. Symp Soc Exp Biol 5:115–129
Perrault C (1680) Essais de Physique. Jean Baptiste Coignard, Paris
Picon C, Guehl J-M, Aussenac G (1996) Growth dynamics, transpiration and water-use efficiency in Quercus robur plants submitted to elevated CO 2 and drought. Ann des Sci For 53:431–446
Plomion C, Bartholomé J, Bouffier L et al (2016) Understanding the genetic bases of adaptation to soil water deficit in trees through the examination of water use efficiency and cavitation resistance: maritime pine as a case study. J Plant Hyd 3:008
Polster H, Weise G, Neuwirth G (1960) Ecological researches on the CO 2 balance [net assimilation] and water economy of some tree species in sand and alkali soils in Hungary. Arch für Forstwes 9:947–1014
Porter R (1979) John Woodward; ‘A droll sort of philosopher’. Geol Mag 116:335–343
Priestley J (1775) Experiments and observations on different kinds of air. J.Johnson, London
Raber O (1937) Water utilization by trees, with special reference to the economic forest species of the north temperate zone. USDA Misc Pub No 257, Washington DC
Ray J (1686) Historia Plantarum, I edn. The Royal Society, London
Ray J (1691) The wisdom of God manifested in the works of creation ; first published in 1691: reprinted by the Wernerian Club, London 1844-1846
Roeser J (1940) The water requirement of Rocky Mountain conifers. J For 38:24–26
Rötzer T, Liao Y, Goergen K, Schüler G, Pretzsch H (2013) Modelling the impact of climate change on the productivity and water-use efficiency of a central European beech forest. Clim Res 58:81–95
Roupsard O, Joly HI, Dreyer E (1998) Variability of initial growth, water-use efficiency and carbon isotope discrimination in seedlings of Faidherbia albida (Del.) A. Chev., a multipurpose tree of semi-arid Africa. provenance and drought effects. Ann des Sci For 55:329–348
Rundel P, Ehleringer J, Nagy K (1989) Stable isotopes in ecological research. Springer-Verlag, New York
Book Google Scholar
Rüsch J (1959) Das Verhältnis von Transpiration und Assimilation als physiologische Kenngröße, untersucht an Pappelklonen. Theor Appl Genet 29:348–354
Scarth GW (1927) Stomatal movement: its regulation and regulatory role - a review. Protoplasma 2:498–511
Schmidt H-L, Winkler F (1979) Einige Ursachen der Variationsbreite von ẟ 13 C-Werten bei C3- und C4-Pflanzen. Ber Dtsch Bot Ges 92:S 185–S 191
Schröder M (1895) The transpiration of various crop plants. Agric For 10:320–336
Shantz HL (1927) Drought resistance and soil moisture. Ecology 8:145–157
Sharrock R (1660) The history of the propagation & improvement of vegetables. A. Lichfield, Oxford
Sinclair TR, Tanner CB, Bennett JM (1984) Water-use efficiency crop production. Bioscience 34:36–40
Sorauer P (1880) Studien über Verdunstung. Wollny - Forschungen auf dem Gebiete der Agrik tom 3:351–490
Spedding J, Ellis R, Heath D (1900) The works of Francis Bacon, Houghton, Mifflin and Company
Stanhill G (1986) John Woodward - a neglected 17th century pioneer of experimental botany. Isr J Bot 35:225–231
Tallec T, Béziat P, Jarosz N, Rivalland V, Ceschia E (2013) Crops’ water use efficiencies in temperate climate: comparison of stand, ecosystem and agronomical approaches. Agric For Meteorol 168:69–81
Tanner CB, Sinclair TR (1983) Efficient water use in crop production: research or re-search? In: Taylor HM, Jordan WR, Sinclair TR (eds) Limitations to efficient water use crop production. American Society of Agronomy, Madison, pp 1–27
Thornthwaite C (1947) Climate and moisture conservation. Ann Assoc Am Geogr 37:87–100
Tomic S, Cussenot M, Dreyer E (2005) La lumiére et les plantes : histoire de la découverte de la « photosynthése », 1779-1804. In: Changeux J-P (ed) La lumière au siècle des lumières et aujourd’hui: Art et science. Odile Jacob, Paris, pp 145–161
Troughton J (1969) Plant water status and carbon dioxide exchange of cotton leaves. Aust J Biol Sci 22:289–302
Tschaplowitz (1878) Ueber die Verdunstung und Substanzzunahme der Pflanzen. Berichte der Sect für Landwirtsch Versuchswes auf der Naturforscherversammlung zu München 1877(tome XX):74
Unger F (1862) Neue Untersuchungen über die Transpiration der Pflanzen. Sitzungsberichte der Kais Akad der Wissenschaften Wien 44:181–217 and 327-368
Vadez V, Kholova J, Medina S, Kakkera A, Anderberg H (2014) Transpiration efficiency: new insights into an old story. J Exp Bot 65:6141–6153
van Helmont J (1662) Oriatrike or Physick Refined. Lodowick Loyyd, London
Vialet-Chabrand S, Matthews JSA, Brendel O, Blatt MR, Wang Y, Hills A, Griffiths H, Rogers S, Lawson T (2016) Modelling water use efficiency in a dynamic environment: an example using Arabidopsis thaliana. Plant Sci 251:65–74
Viets FG (1962) Fertilizers and the efficient use of water. Adv Agron 14:223–264
Virgona J, Hubick K, Rawson H et al (1990) Genotypic variation in transpiration efficiency, carbon-isotype discrimination and carbon allocation during early growth in sunflower. Aust J Plant Physiol 17:207–214
Vivas M, Rolo V, Wingfield MJ, Slippers B (2019) Maternal environment regulates morphological and physiological traits in Eucalyptus grandis . For Ecol Man 432:631–636
Vogel JC (1980) Fractionation of the carbon isotopes during photosynthesis. In: Sitzungsberichte der Heidelberger Akademie der Wissenschaften. Springer, New York, pp 111–135
von Höhnel FR (1879) Ueber die Wasserverbrauchsmengen unserer Forstbäume mit Beziehung auf die forstlich-meteorologischen Verhältnisse. Wollny - Forschungen aus dem Gebiet der Agric tom II:398–421
von Schleiden MJ (1849) Grundzüge der wissenschaftlichen Botanik, 3rd edn. Verlag von Wilhelm Engelmann, Leipzig
von Seelhorst C (1902) Vegetationskästen zum Studium des Wasserhaushaltes im Boden. J Landwirtsch 50:277–280
Woodward J (1699) Some thoughts and experiments concerning vegetation. Philos Trans R Soc Lond A 21:193–227
Zhang J, Marshall JD (1994) Population differences in water-use efficiency of well-watered and water-stressed western larch seedlings. Can J For Res 24:92–99
Download references
Acknowledgements
Much of the historical background is based on A.G. Morton’s “History of Botanical Sciences” as well as to Frank N. Egerton’s “A History of the Ecological Sciences” series in the “Bulletin of the Ecological Society of America”. The author is also largely indebted to C. Schuchardt from the Library of the Staatsbetrieb Sachsenforst for help with the quest for rare German publications. The author would also like to thank E. Dreyer and J.M. Guehl (both from the SILVA Unit at INRAE Nancy, France) who commented extensively on an earlier version of the draft and J. Williams (University of Sussex), L. Handley and J. Raven (University of Dundee) who made many valuable suggestions and improved language.
Author information
Authors and affiliations.
Université de Lorraine, AgroParisTech, INRAE, UMR Silva, Nancy, France
Oliver Brendel
You can also search for this author in PubMed Google Scholar
Corresponding author
Correspondence to Oliver Brendel .
Ethics declarations
Conflict of interest.
The author declares no competing interests.
Additional information
Handling Editor: Andrew Merchant
Publisher’s note
Springer Nature remains neutral with regard to jurisdictional claims in published maps and institutional affiliations.
The original online version of this article was revised due to a retrospective Open Access order.
Rights and permissions
Open Access This article is licensed under a Creative Commons Attribution 4.0 International License, which permits use, sharing, adaptation, distribution and reproduction in any medium or format, as long as you give appropriate credit to the original author(s) and the source, provide a link to the Creative Commons licence, and indicate if changes were made. The images or other third party material in this article are included in the article's Creative Commons licence, unless indicated otherwise in a credit line to the material. If material is not included in the article's Creative Commons licence and your intended use is not permitted by statutory regulation or exceeds the permitted use, you will need to obtain permission directly from the copyright holder. To view a copy of this licence, visit http://creativecommons.org/licenses/by/4.0/ .
Reprints and permissions
About this article
Cite this article.
Brendel, O. The relationship between plant growth and water consumption: a history from the classical four elements to modern stable isotopes. Annals of Forest Science 78 , 47 (2021). https://doi.org/10.1007/s13595-021-01063-2
Download citation
Received : 26 November 2020
Accepted : 14 April 2021
Published : 28 April 2021
DOI : https://doi.org/10.1007/s13595-021-01063-2
Share this article
Anyone you share the following link with will be able to read this content:
Sorry, a shareable link is not currently available for this article.
Provided by the Springer Nature SharedIt content-sharing initiative
- Transpiration efficiency
- Water use efficiency
- Plant ecophysiology
- Botanical history
Annals of Forest Science
ISSN: 1297-966X
- Submission enquiries: [email protected]
- General enquiries: [email protected]
Advertisement
Research Progress on endangered plants: a bibliometric analysis
- Review Paper
- Published: 07 March 2022
- Volume 31 , pages 1125–1147, ( 2022 )
Cite this article
- Jie Xu 1 , 3 ,
- Pengnan Xiao 2 ,
- TingTing Li 1 , 3 &
- Zhengxiang Wang ORCID: orcid.org/0000-0001-9514-6712 1 , 3
902 Accesses
10 Citations
Explore all metrics
The rapid extinction of endangered plants (EPs) may lead to the destruction of entire ecosystems, which will seriously threaten the survival and development of humans. Research on endangered plants should be strengthened to scientifically guide the protection of endangered plants. Based on 1635 publications collected from the Web of Science Core Collection ™ (WoS), this paper aims to provide a comprehensive bibliometric run-through and visualization of the subject of EPs. Contingent on influential authors, organizations, top journals and subject category, as well as the most influential papers in the EPs field were discussed in detail. Afterwards, by analyzing the co-occurrence network and evolution path of keywords, to discover the main research topics. In the discussion part, this paper focuses on important issues and research frameworks in the field. The main motive of the paper is to assist research workers interested in the area of EPs to determine the ongoing potential research opportunities and hotspots.
This is a preview of subscription content, log in via an institution to check access.
Access this article
Price includes VAT (Russian Federation)
Instant access to the full article PDF.
Rent this article via DeepDyve
Institutional subscriptions
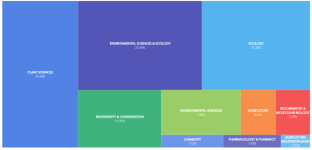
Similar content being viewed by others
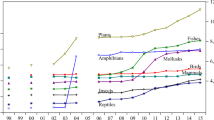
Impact of climate change on biodiversity loss: global evidence
Muzafar Shah Habibullah, Badariah Haji Din, … Hasan Zahid
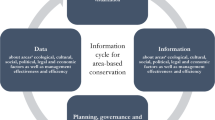
Challenges and opportunities of area-based conservation in reaching biodiversity and sustainability goals
Samuel Hoffmann
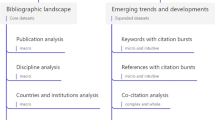
Ecotourism and sustainable development: a scientometric review of global research trends
Lishan Xu, Changlin Ao, … Zhenyu Cai
Data Availability
Not applicable.
Code Availability
Adhikari D, Barik SK, Upadhaya K (2012) Habitat distribution modelling for reintroduction of Ilex khasiana Purk., a critically endangered tree species of northeastern India. Ecol Eng 40:37–43. https://doi.org/10.1016/j.ecoleng.2011.12.004
Article Google Scholar
Bacles CFE, Lowe AJ, Ennos RA (2004) Genetic effects of chronic habitat fragmentation on tree species: the case of Sorbus aucuparia in a deforested Scottish landscape. Mol Ecol 13, 573-584.10.1046/j.1365-294X.2004.02093.x
Barrett S, Kohn JR (1991) Genetic and Evolutionary Consequences of Small Population Size in Plants: Implications for Conservation. Genetics & Conservation of Rare Plants
Caro TM, O. G (1999) On the Use of Surrogate Species in Conservation Biology.Conserv Biol(4),805–814
Caro T, Laurenson M, Karen M (1994) Ecological and genetic factors in conservation: A cautionary tale. Science
Chao S, Wang S, Xia EH, Jiang JJ, Zeng FC, Gao LZ (2016) Full transcription of the chloroplast genome in photosynthetic eukaryotes. Sci Rep-Uk.10.1038/srep30135
Chen L, Gao QK, Chen DM, Xu CJ (2005) The use of RAPD markers for detecting genetic diversity, relationship and molecular identification of Chinese elite tea genetic resources [Camellia sinensis (L.) O. Biodivers Conserv 14:1433–1444
CM C (2006) CiteSpace II: Detecting and visualizing emerging trends and transient patterns in scientific literature. Journal of the American Society for Information Science and Technology (3),359–377. 10.1002/asi.20317
Csergő AM, Salguero Gómez R, Broennimann O, Coutts SR, Guisan A, Angert AL, Welk E, Stott I, Enquist BJ, McGill B, Svenning JC, Violle C, Buckley YM (2017) Less favourable climates constrain demographic strategies in plants. Ecol Lett 20, 969-980.10.1111/ele.12794
Cyranoski D (2008) China: Visions of China. Nature 454, 384-387.10.1038/454384a
Dongyan X (2004) A floristic study of the rare and endangered plants in Baimashan nature reserve. JOURNAL OF HAINAN NORMAL UNIVERSITY 17, 278–281, 302.10.3969/j.issn.1674-4942.2004.03.017
Draper D, Rosselló-Graell A, Garcia C, Gomes CT, Sérgio (2003) C., Application of GIS in plant conservation programmes in Portugal. Biol Conserv 113, 337–349
Tao DU (2017) W. S. Q. X., Analysis of competitiveness of international geographic institutes based on bibliometrics. Acta Geographica Sinica 72, 1702-1716.10.11821/dlxb201709014
Du Xiajin, Hengan Z, Xiaofeng L, Li W (2017) Megasporogenesis and Development of Female Gametophyte of Endangered Plant Diplopanax stachyanthus. Journal of Tropical and Subtropical Botany 25, 605-609.10.11926/jtsb.3696
Eck N, Waltman L (2010) Software survey: VOSviewer, a computer program for bibliometric mapping. Scientometrics 84:523–538
Eggleton LHJB (1998) Biodiversity inventories, indicator taxa and effects of habitat modification in tropical forest. Nature
El-Banhawy A, Nour IH, Acedo C, Elkordy A, Ellmouni Y (2021) Taxonomic Revisiting and Phylogenetic Placement of Two Endangered Plant Species: Silene leucophylla Boiss. and Silene schimperiana Boiss. (Caryophyllaceae) Plants 10:740
Article CAS Google Scholar
Elith J, Graham H, Anderson CP, Dudík R, Ferrier M, Guisan S, Hijmans AJ, Huettmann R, Leathwick FR, Lehmann J, Li A, Lohmann JG, Loiselle LA, Manion B, Moritz G, Nakamura C, Nakazawa M, Overton YMcCM, Townsend Peterson J, Phillips AJ, Richardson S, Scachetti-Pereira K, Schapire RE, Soberón R, Williams J, Wisz SS, Zimmermann ME (2006) N., Novel methods improve prediction of species’ distributions from occurrence data. Ecography 29, 129-151.10.1111/j.2006.0906-7590.04596.x
Engelmann F (2011) Use of biotechnologies for the conservation of plant biodiversity. In Vitro Cellular & Developmental Biology - Plant 47, 5-16.10.1007/s11627-010-9327-2
Fanrong F (2008) Research on Endangered Plant Baiguimu’s Endangered System and Ex-situ Conservation. Fujian Agriculture and Forestry University
Fanrong F, Xiangqing M, Mark P (2008) Research progress in conservation biology of endangered plants in China. CHINA FORESTRY SCIENCE AND TECHNOLOGY, pp 1–5
Farwig N, Braun C, Hning-Gaese B (2008) K., Human disturbance reduces genetic diversity of an endangered tropical tree, Prunus africana (Rosaceae). Conserv Genet 9, 317.10.1007/s10592-007-9343-x
Fenu G, Bacchetta G, Charalambos SC, Fournaraki C, Galdo G, Gotsiou P, Kyratzis A, Piazza C, Vicens M, Pinna MS (2019) An early evaluation of translocation actions for endangered plant species on Mediterranean islands. Plant Diversity 41, 94-104.10.1016/j.pld.2019.03.001
Garfield E, Paris SW, Stock WG (2006) HistCite: A software tool for informetric analysis of citation linkage. NFD Information-Wissenschaft und Praxis 57:391–400
Google Scholar
Gibbs JP (2001) Demography versus habitat fragmentation as determinants of genetic variation in wild populations. Biol Conserv 100, 15-20.10.1016/S0006-3207(00)00203-2
Godefroid S, Piazza C, Rossi G, Buord S, Stevens A, Aguraiuja R, Cowell C, Weekley CW, Vogg G, Iriondo JM, Johnson I, Dixon B, Gordon D, Magnanon S, Valentin B, Bjureke K, Koopman R, Vicens M, Virevaire M, Vanderborght T (2011) How successful are plant species reintroductions? Biol Conserv 144, 672-682.10.1016/j.biocon.2010.10.003
Goodman BD (1990) The demography of chance extinction. Viable Population for Conservation
Guirui Y (2003) Global Change and Carbon Cycle and Carbon Storage in Terrestrial Ecosystem (Fine). Meteorological Press
Guisan A, Broennimann O, Engler R (2006) Using Niche-Based Models to Improve the Sampling of Rare Species. Conserv Biol 20, 501-511.10.1111/j.1523-1739.2006.00354.x
Guisan A, Zimmermann NE (2000) Predictive habitat distribution models in ecology. Ecol Model 135, 147-186.10.1016/S0304-3800(00)00354-9
Guo-hua L, Bo-jie F (2001) Effects of global climate change on forest ecosystems. JOURNAL OF NATURAL RESOURCES, 71-78.10.3321/j.issn:1000-3037.2001.01.013
Honnay O, Jacquemyn H (2007) Susceptibility of Common and Rare Plant Species to the Genetic Consequences of Habitat Fragmentation. Conserv Biol 21, 823-831.10.1111/j.1523-1739.2006.00646.x
Huang J, Chen B, Liu C, Lai J, Zhang J, Ma K (2012) Identifying hotspots of endemic woody seed plant diversity in China. Divers Distrib 18, 673-688.10.1111/j.1472-4642.2011.00845.x
IUCN (2021) IUCN Red List of Threatened Species. IUCN
Jeong HM, Kim HR, Hong S, You YH (2018) Effects of elevated CO2 concentration and increased temperature on leaf quality responses of rare and endangered plants. Journal of Ecology & Environment 42, 1.10.1186/s41610-017-0061-0
Jiakuan HSGY (1998) Variation of flower number and seed setting rate of Liriodendron tulipifera. Acta Botanica Sinica, 27-32.10.3321/j.issn:1672-9072.1998.01.003
Jin’E M (2008) Study on Physiological and Ecological Characteristics of Endangered Plant Sinocalycanthus. Southwest University
Jingyun F (2000) Global Ecology: Climate Change and Ecological Response
Lande MR (1991) Assessing Extinction Threats: Toward a Reevaluation of IUCN Threatened Species Categories. Conserv Biol 5:148–157
Legname G, Nguyen HOB, Baskakov IV, Cohen FE, DeArmond SJ, Prusiner SB (2005) Strain-specified characteristics of mouse synthetic prions. Proceedings of the National Academy of Sciences 102, 2168-2173.10.1073/pnas.0409079102
Liang F, Huang Q, Yanping YU, Liang H, Huang Q, Liu X, Chen Q, Tan X, University YN (2019) Physiological response of endangered semi-mangrove Barringtonia racemosa to salt stress and its correlation analysis. Journal of Central South University of Forestry & Technology 39, 12-18.10.14067/j.cnki.1673-923x.2019.10.003
Li-hua Z, Xiu-zhen C, Hong-liang Z (2006) Endangering Mechanism and Strategy for Conservation of Endangered Plant. Journal of Hunan Institute of Humanities, Science and Technology, pp 43–46. 10.3969/j.issn.1673-0712.2006.06.015
Liu H, Yan MA, Qichao WU, Wang Y, Zang D, University SA (2020) Intraspecific and interspecific competition of the endangered plant Salix taishanensis. Journal of Forest and Environment 40, 178-183.10.13324/j.cnki.jfcf.2020.02.010
Liu N, Zhu W, Sun Z, Yang L, Yuan S, Ren H (2014) Canopy Size Dependent Facilitations from the Native ShrubRhodomyrtus tomentosa to the Early Establishment of Native TreesCastanopsis fissa and Syzygium hancei in Tropical China. Restor Ecol 22, 509-516.10.1111/rec.12094
Liu Z, Shu Q, Wang L, Yu M, Hu Y, Zhang H, Tao Y, Shao Y (2012) Genetic diversity of the endangered and medically important Lycium ruthenicum Murr. revealed by sequence-related amplified polymorphism (SRAP) markers. Biochem Syst Ecol 45, 86-97.10.1016/j.bse.2012.07.017
Liwen W (2016) The value of U.S. national parks. Grand Garden of Science, 3.10.3969/j.issn.1003-1871.2016.22.007
Mark FFMX (2008) Research progress in conservation biology of endangered plants in China. CHINA FORESTRY SCIENCE AND TECHNOLOGY, 1-5.10.3969/j.issn.1000-8101.2008.03.001
Myers N (1988) Myers N. — Threatened biotas: “Hot Spots” in tropical forests. Environmentalist The Environmentalist 8:187–208
Nicotra A, Hoyle G, Steadman K, Good R (2014) Australian alpine seed ecology: plant conservation and adaptation to climate change. ARC linkage project, Symposium on Alpine Seed & Plant Ecology in the Australian Alps
Ning X, Chen LN, Khoon-Meng W, Cui YZ, Yang HQ, Monica S (2016) Seed Set and Natural Regeneration of Dendrocalamus membranaceus Munro after Mass and Sporadic Flowering in Yunnan, China. Plos One 11, e153845.10.1371/journal.pone.0153845
Olde Venterink H, van der Vliet RE, Wassen MJ (2001) Nutrient limitation along a productivity gradient in wet meadows. Plant Soil 234, 171-179.10.1023/A:1017922715903
Orme CDL, Davies RG, Burgess M, Eigenbrod F, Pickup N, Olson VA, Webster AJ, Ding T, Rasmussen PC, Ridgely RS, Stattersfield AJ, Bennett PM, Blackburn TM, Gaston KJ (2005a) Owens, I. P. F., Global hotspots of species richness are not congruent with endemism or threat. Nature 436, 1016-1019.10.1038/nature03850
Orme CDL, Davies RG, Burgess M, Eigenbrod F, Pickup N, Olson VA, Webster AJ, Ding T, Rasmussen PC, Ridgely RS, Stattersfield AJ, Bennett PM, Blackburn TM, Gaston KJ (2005b) Owens, I. P. F., Global hotspots of species richness are not congruent with endemism or threat. Nature 436, 1016-1019.10.1038/nature03850
Petrie M, Bradford D, Hubbard JB, Lauenroth RM, Andrews WK (2017) C., M., Climate change may restrict dryland forest regeneration in the 21st century. Ecology.10.1002/ecy.1791
Quanguang Y, Chai S, Wu R, Zou R, Wei X (2020) Characteristics of Concomitant Community and Population Structure of Endangered Plant Camellia tunghinensis. Guangxi Forestry Science 036, 45–55, 64.10.3969/j.issn.1006-1126.2020.04.003
Rovzar C, Gillespie TW, Kawelo K (2016) Landscape to site variations in species distribution models for endangered plants. Forest Ecol Manag 369, 20-28.10.1016/j.foreco.2016.03.030
Saccheri I, Kuussaari M, Kankare M, Vikman P, Fortelius W, Hanski (1998) I., Inbreeding and extinction in a butterfly metapopulation. Nature 392, 491
Salmerón-Sánchez E, Mendoza-Fernández AJ, Lorite J, Mota JF, Peñas J (2021) Plant conservation in Mediterranean-type ecosystems. Mediterranean Botany 42, e71333.10.5209/mbot.71333
Sarasan V, Cripps R, Ramsay MM, Atherton C, McMichen M, Prendergast G, Rowntree JK (2006) CONSERVATION IN VITRO OF THREATENED PLANTS – PROGRESS IN THE PAST DECADE. In vitro cellular & developmental biology. Plant 42, 206-214.10.1079/IVP2006769
Shao-xian W, Fei W, Cheng-bai L, Zhi-yong Y, Xia C (2008) Application of DNA molecular markers in endangered species conservation.CHINESE JOURNAL OF ECOLOGY,250–256.CNKI:SUN:STXZ.0.2008-02-021
Shengce T, Zhiping Z, Xianen Z (2001) Development of PCR Technology. China Biotechnology 21, 26-29.10.3969/j.issn.1671-8135.2001.04.006
Song G, Haiqun W, Canming Z, Deyuan H (1997) Genetic Diversity and Population Differentiation of Cathaya Argyrophylla in Bamian Mountain. J Integr Plant Biol , 266–271
Stephen B, See-Chung C, Chong SL, Frieda C, Fiona I, Putri WU, Neil W, Wortley AH (2012) Observations on the Morphology, Pollination and Cultivation of Coco de Mer (Lodoicea maldivica (J F Gmel.) Pers., Palmae). Journal of Botany,2012,(2012-3-14) 2012, 1-13.10.1155/2012/687832
Steven S (2013) Plant Reintroduction in a Changing Climate: Promises and Perils. Restor Ecol
Sumin LI, You W, Xiao S, Dongjin HE, Hong W, Liu J (2015) Gap micro-environment of endangered plant Tsuga longibracteata forests in Tianbaoyan National Nature Reserve. Journal of Forest and Environment 35.10.13324/j.cnki.jfcf.2015.04.009
Sun J, Wu Y, He Y (2018) The Geographical Sciences during 1986–2015: From the Classics to the Frontiers
Tingting LCLP (2009) The Niche Characteristics of Endangered Plant Tsuga tchekiangensisin Jiulongshan National Nature Reserve of Zhejiang Province. JOURNAL OF WUHAN BOTANICAL RESEARCH 27, 55-61.10.3969/j.issn.2095-0837.2009.01.009
van Eck NJ, Waltman L (2010) Software survey: VOSviewer, a computer program for bibliometric mapping. Scientometrics 84, 523-538.10.1007/s11192-009-0146-3
Vitousek PM (1994) Beyond Global Warming: Ecology and Global Change. Ecology 75, 1861-1876.10.2307/1941591
Volis S (2016) How to conserve threatened Chinese plant species with extremely small populations? Plant Diversity 38, 45-52.10.1016/j.pld.2016.05.003
Wassen MJ, Venterink HO, Lapshina ED, Tanneberger F (2005) Endangered plants persist under phosphorus limitation. Nature 437, 547-550.10.1038/nature03950
Wassen M, Venterink H, Lapshina E, Tanneberger F (2005) Endangered plants persist under phosphorus limitation. Nature 437, 547-550.10.1038/nature03950
Wei L, Guihua L, Jin Z, Desi (2002) H.,
Studies on the Seed Bank of Freshwater Wetland:A review. ACTA ECOLOGICA SINICA, 395-402.10.3321/j.issn:1000-0933 (2002) 03.016
Whittaker RJ, Araújo MB, Jepson P, Ladle RJ, Watson JEM, Willis KJ (2005) Conservation Biogeography: assessment and prospect. Divers Distrib 11, 3-23.10.1111/j.1366-9516.2005.00143.x
Wilson EO (1988) Biodiversity, National Forum on Biodiversity, Washington D.C. 1986
Xianwei Y, Jie C, Ge, Ying, Guan, Baohua F, Meiying, Qiu Y (2002) A comparison of dormancy and germination of seeds between an endangered species, Changium smyrnioides, and a non-endangered species, Anthriscus sylvestris. Biodivers Sci 10:425–430
Xiao-xue CSYX (2010) Seed germination characteristics of endangered plant Sinia rhodoleuca. Chin J Ecol 29:233–237. doi: https://doi.org/10.7498/aps.62.174102
Xibin ZSBX (2014) Progress in Chinese geography research reflected from Acta Geographica Sinica during 1934–2013:A bibliometrics analysis. Acta Geographica Sinica 69, 1077-1092.10.11821/dlxb201408005
Xin-Hua ZDJ (1999) Progress in studies of genetic diversity and conservation biology of endangered plant species. CHINESE BIODIVERSITY, 31-37.10.3321/j.issn:1005-0094.1999.01.006
Xin-ran W (2015) Application and Prospect of Biotechnology in the Research and Development of Medicinal Plants. Science&Technology Vis 46:2343
Xu L (2017) The application and prospect of biotechnology in the research and development of medicinal plants. REGIONAL GOVENANCE (5).10.3969/j.issn.2096-4595.2017.05.134
YAN ZU YT (1998) ECOLOGICAL ANALYSIS ON SEXUAL REPRODUCTIVE PRODUCE AND ENDANGERED REASON OF RHODIOLA SACHALINENSIS. Plant Res 18:336–340
Yinghong J (2018) Analysis of the ecological characteristics and endangered causes of the extremely small population of Phoebe hainanensis. Central South University of Forestry and Technology
Yong ZYLY (2017) Flower phenology and breeding system of endangered mangrove Lumnitzera littorea (Jack.) Voigt. Chinese Journal of Applied & Environmental Biology 23, 77-81.10.3724/SP.J.1145.2016.03021
Yuanhuo D, Zhou S (2008) The classification of endangered degrees of species and the progress in researches on species- endangering mechanisms. BIOLOGY TEACHING 33, 5-6.10.3969/j.issn.1004-7549.2008.06.002
Ze-xin J, Jun-min L (2007) ISSR analysis on genetic diversity of endangered relic shrub Sinocalycanthus chinensis. Chin J Appl Ecol 18:247–253
Zhihuan H (2020a) The Research Progress of Endangered Causes and Protection Strategy of Rare and Endangered Plants in China. Journal of University of Souht China(Science & Technology) 34, 42-50.10.19431/j.cnki.1673-0062.2020.03.007
Zhihuan H (2020b) The Research Progress of Endangered Causes and Protection Strategy of Rare and Endangered Plants in China. J Univ Souht China(Science Technology) 34:42 -50.10. 19431 /j. cnki
Zhuang XLGT (2011) Pollination biology of Camellia changii. Chinese Journal of Ecology 30, 552-557.10.3724/SP.J.1011.2011.00150
Zimmermann NE, Yoccoz NG, Edwards TC, Meier ES, Thuiller W, Guisan A, Schmatz DR, Pearman PB (2009) Climatic extremes improve predictions of spatial patterns of tree species.P Natl Acad Sci Usa106
Download references
This research was funded by the National Natural Science Foundation of China (42101065), the Central Government Guides Local Science and Technology Development Projects(2019ZYYD050), the Open Foundation of Regional Development and Environmental Response, Key Laboratory of Hubei Province (2021(C)002.)
Author information
Authors and affiliations.
Faculty of Resources and Environmental Science, Hubei University, 430062, Wuhan, China
Jie Xu, TingTing Li & Zhengxiang Wang
The College of Urban & Environmental Sciences, Central China Normal University, 430079, Wuhan, China
Pengnan Xiao
Regional Development and Environmental Response, Key Laboratory of Hubei Province, 430062, Wuhan, China
You can also search for this author in PubMed Google Scholar
Contributions
Conceptualization, ZX.W and J.X.; methodology, J.X and P.X.; software, P.X. and J.X and TT.L.; writing—original draft preparation, P.X. All authors have read and agreed to the published version of the manuscript.”
Corresponding author
Correspondence to Zhengxiang Wang .
Ethics declarations
Statements and declarations.
The authors declare that they have no known competing financial interests or personal relationships that could have appeared to influence the work reported in this paper.
Conflict of interest
The authors declare no conflicts of interest.
Additional information
Communicated by Daniel Sanchez Mata.
Publisher’s Note
Springer Nature remains neutral with regard to jurisdictional claims in published maps and institutional affiliations.
Rights and permissions
Reprints and permissions
About this article
Xu, J., Xiao, P., Li, T. et al. Research Progress on endangered plants: a bibliometric analysis. Biodivers Conserv 31 , 1125–1147 (2022). https://doi.org/10.1007/s10531-022-02392-y
Download citation
Received : 20 June 2021
Revised : 16 January 2022
Accepted : 25 January 2022
Published : 07 March 2022
Issue Date : March 2022
DOI : https://doi.org/10.1007/s10531-022-02392-y
Share this article
Anyone you share the following link with will be able to read this content:
Sorry, a shareable link is not currently available for this article.
Provided by the Springer Nature SharedIt content-sharing initiative
- Research hotspots
- Conservation
- Bibliometrics
- Endangered system
- Find a journal
- Publish with us
- Track your research
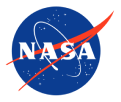
Suggested Searches
- Climate Change
- Expedition 64
- Mars perseverance
- SpaceX Crew-2
- International Space Station
- View All Topics A-Z
Humans in Space
Earth & climate, the solar system, the universe, aeronautics, learning resources, news & events.
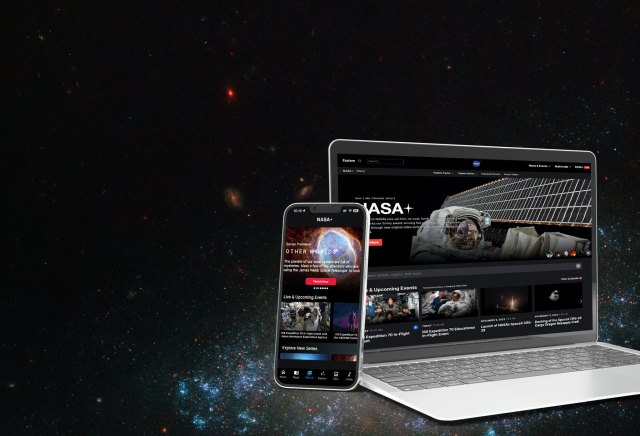
NASA Receives 13 Nominations for the 28th Annual Webby Awards
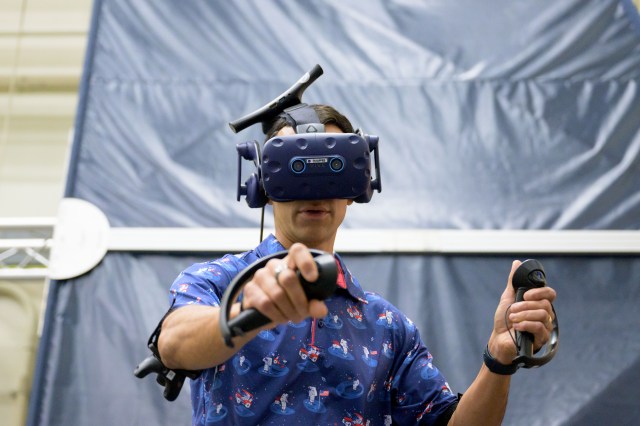
Through Astronaut Eyes, Virtual Reality Propels Gateway Forward
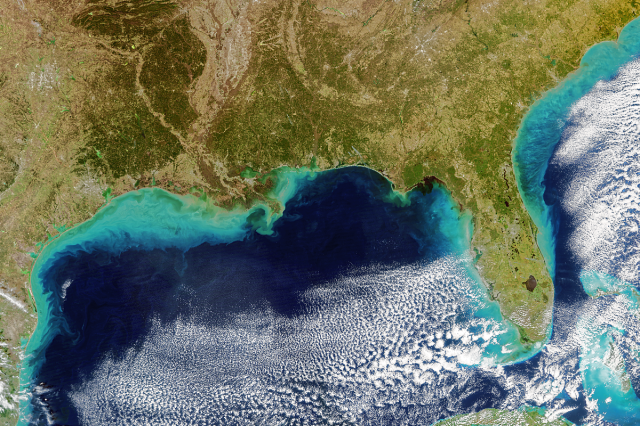
How NASA Spotted El Niño Changing the Saltiness of Coastal Waters
- Search All NASA Missions
- A to Z List of Missions
- Upcoming Launches and Landings
- Spaceships and Rockets
- Communicating with Missions
- James Webb Space Telescope
- Hubble Space Telescope
- Why Go to Space
- Astronauts Home
- Commercial Space
- Destinations
- Living in Space
- Explore Earth Science
- Earth, Our Planet
- Earth Science in Action
- Earth Multimedia
- Earth Science Researchers
- Pluto & Dwarf Planets
- Asteroids, Comets & Meteors
- The Kuiper Belt
- The Oort Cloud
- Skywatching
- The Search for Life in the Universe
- Black Holes
- The Big Bang
- Dark Energy & Dark Matter
- Earth Science
- Planetary Science
- Astrophysics & Space Science
- The Sun & Heliophysics
- Biological & Physical Sciences
- Lunar Science
- Citizen Science
- Astromaterials
- Aeronautics Research
- Human Space Travel Research
- Science in the Air
- NASA Aircraft
- Flight Innovation
- Supersonic Flight
- Air Traffic Solutions
- Green Aviation Tech
- Drones & You
- Technology Transfer & Spinoffs
- Space Travel Technology
- Technology Living in Space
- Manufacturing and Materials
- Science Instruments
- For Kids and Students
- For Educators
- For Colleges and Universities
- For Professionals
- Science for Everyone
- Requests for Exhibits, Artifacts, or Speakers
- STEM Engagement at NASA
- NASA's Impacts
- Centers and Facilities
- Directorates
- Organizations
- People of NASA
- Internships
- Our History
- Doing Business with NASA
- Get Involved
- Aeronáutica
- Ciencias Terrestres
- Sistema Solar
- All NASA News
- Video Series on NASA+
- Newsletters
- Social Media
- Media Resources
- Upcoming Launches & Landings
- Virtual Events
- Sounds and Ringtones
- Interactives
- STEM Multimedia
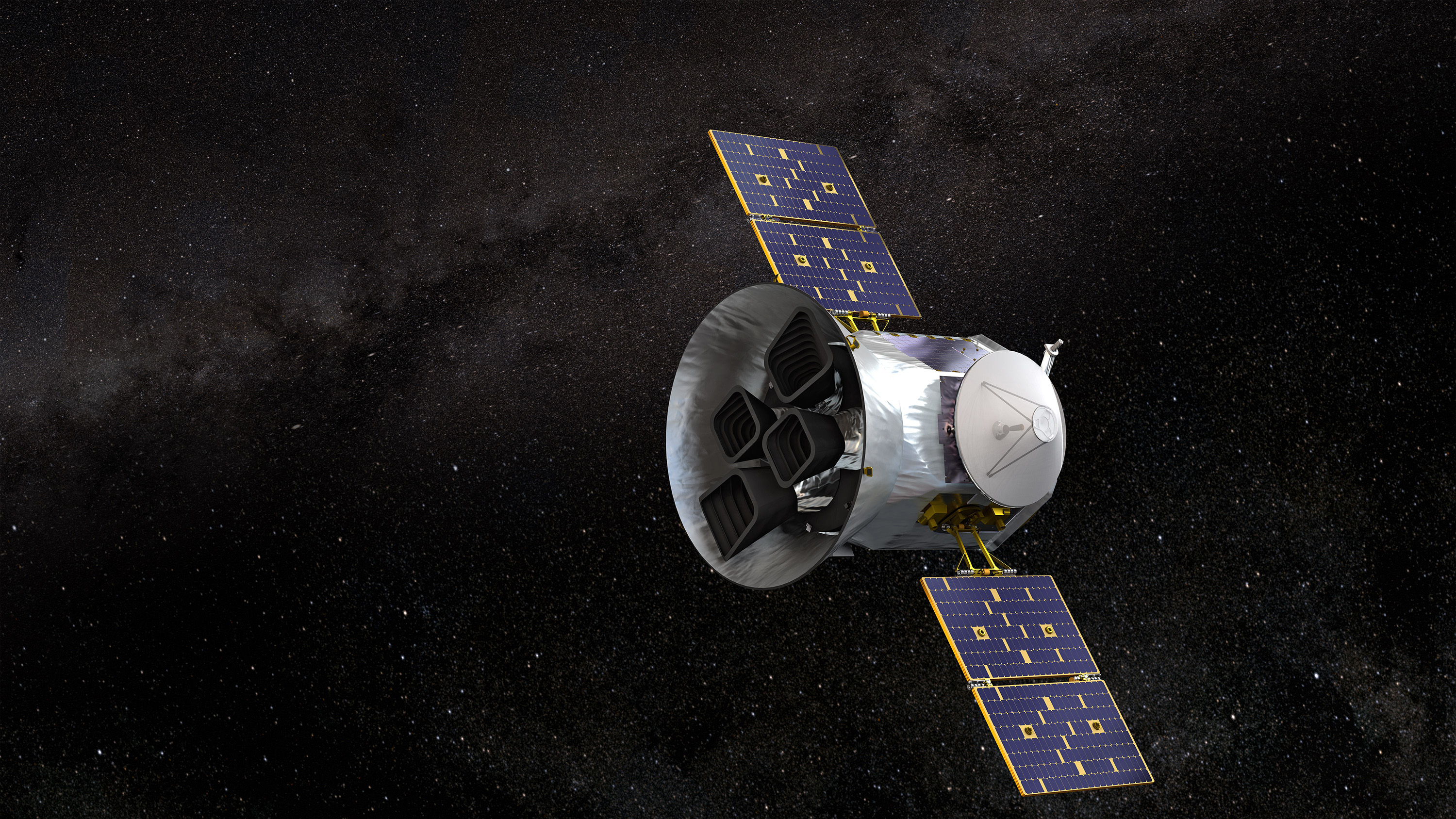
NASA’s TESS Temporarily Pauses Science Observations
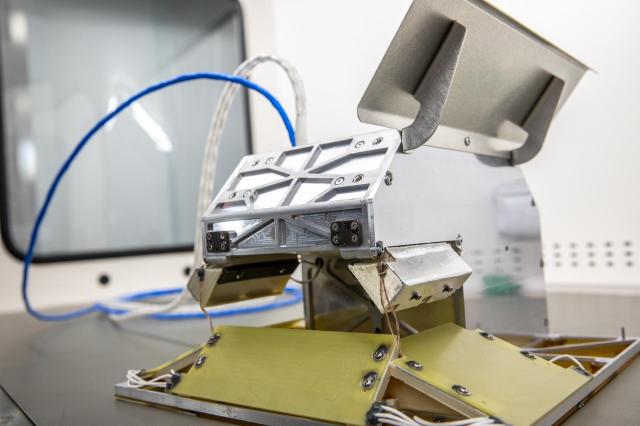
NASA Technology Helps Guard Against Lunar Dust
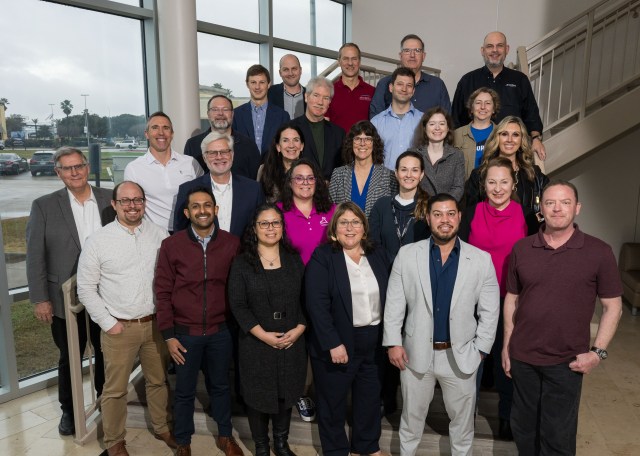
NASA Shares Medical Expertise with New Space Station Partners
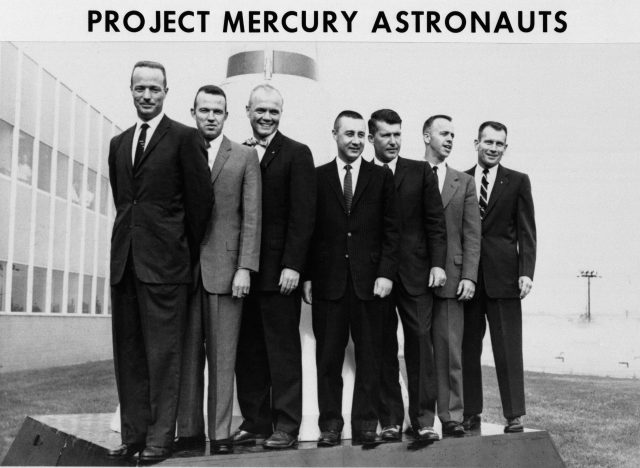
From NASA’s First Astronaut Class to Artemis II: The Importance of Military Jet Pilot Experience
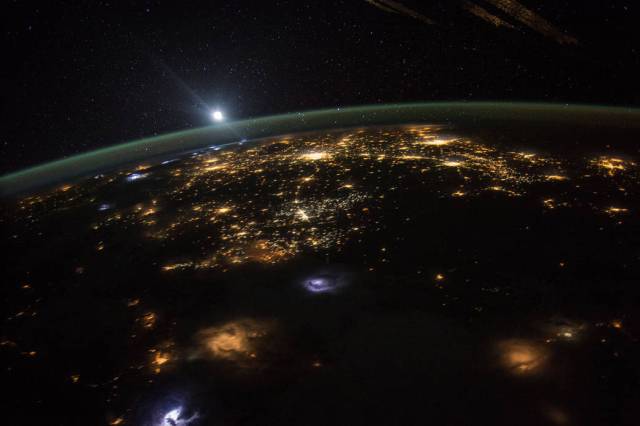
Commercial Space Frequently Asked Questions
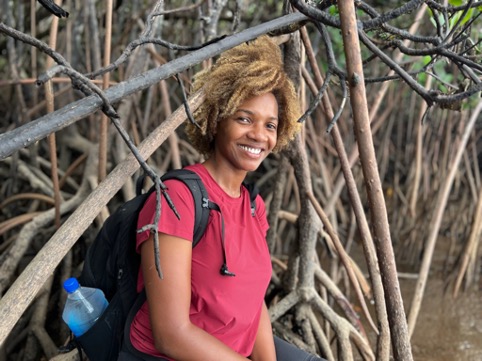
NASA’s Lola Fatoyinbo Receives Royal Geographical Society Prize
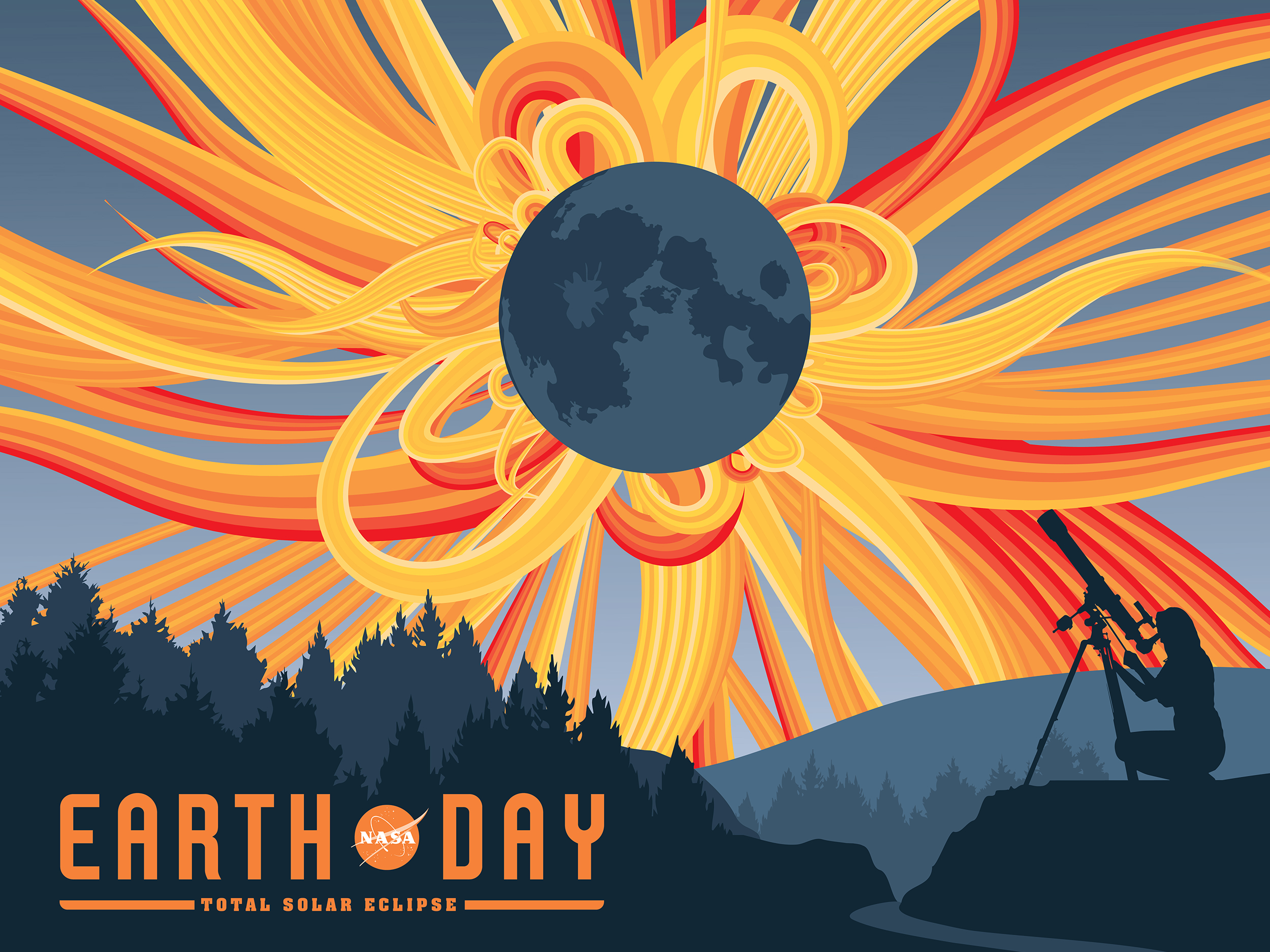
Earth Day Toolkit
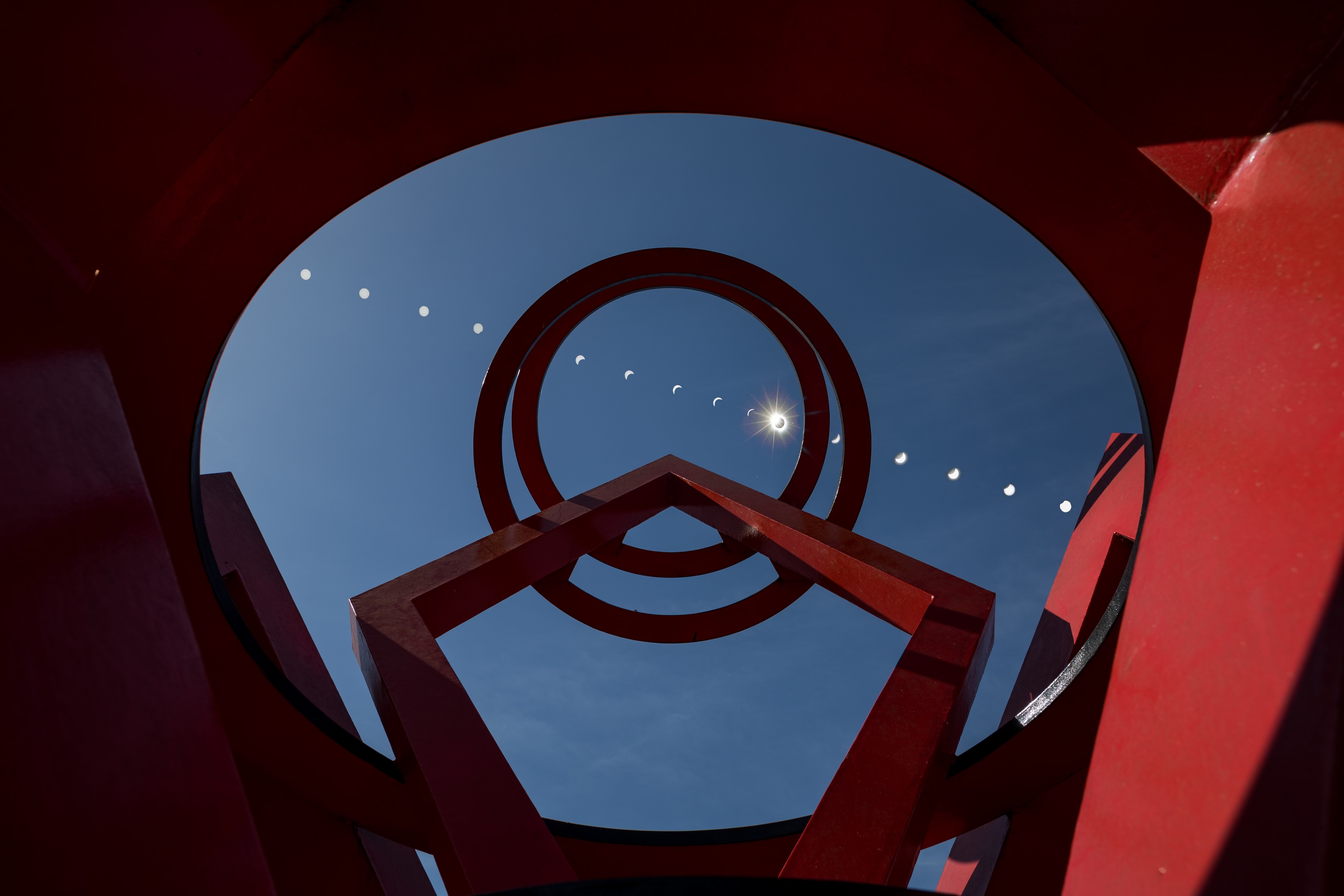
More Than 36,000 Volunteers Helped Do NASA Eclipse Science
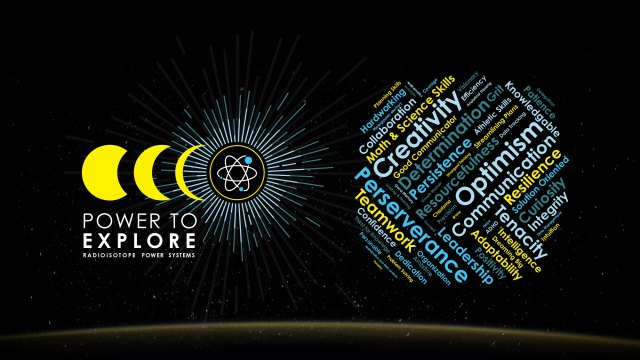
NASA Names Finalists of the Power to Explore Challenge
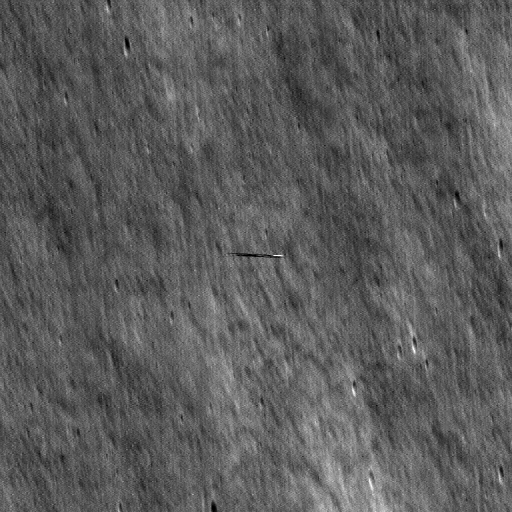
NASA’s LRO Finds Photo Op as It Zips Past SKorea’s Danuri Moon Orbiter
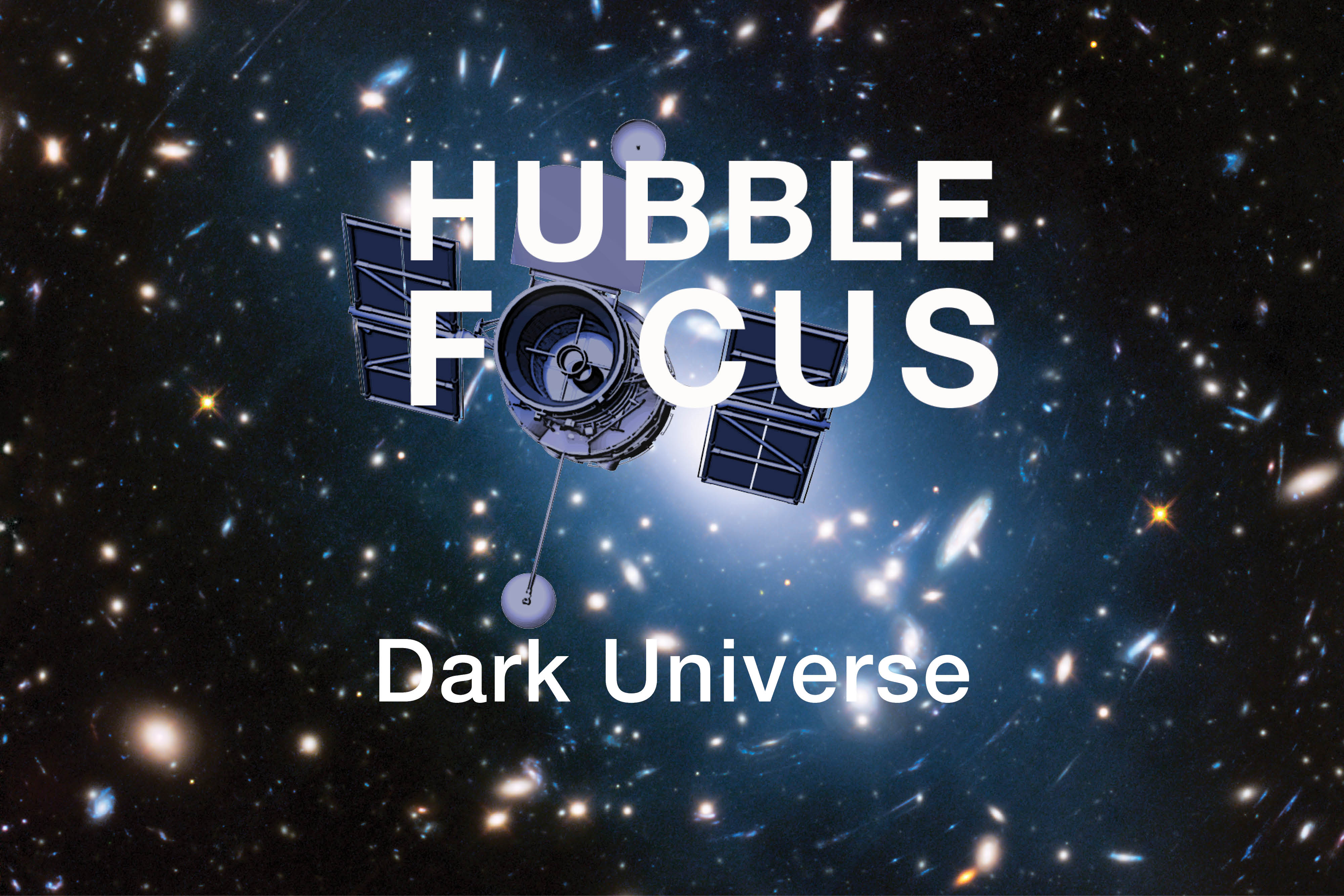
NASA’s New Hubble E-Book Spotlights Universe’s Best-Kept Dark Secrets
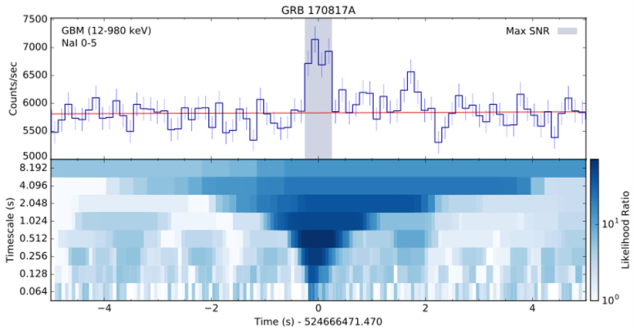
SRPD Gamma-ray Astrophysics
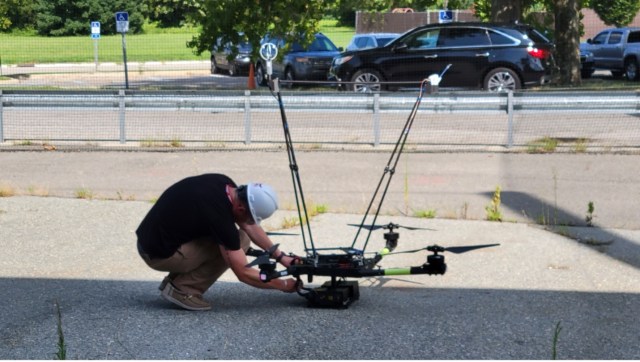
NASA Langley Team to Study Weather During Eclipse Using Uncrewed Vehicles
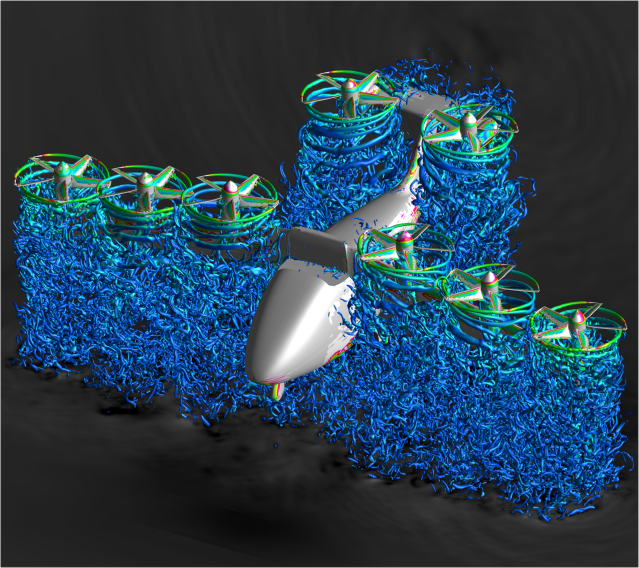
NASA Noise Prediction Tool Supports Users in Air Taxi Industry
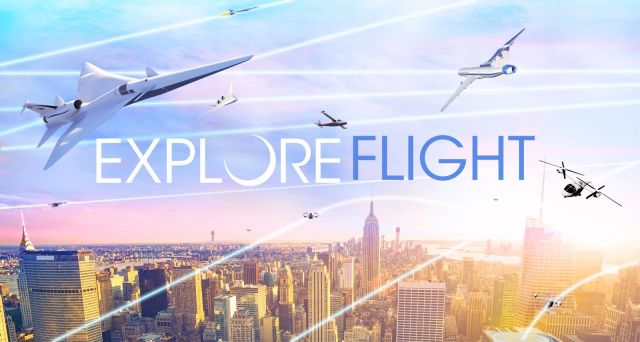
ARMD Solicitations
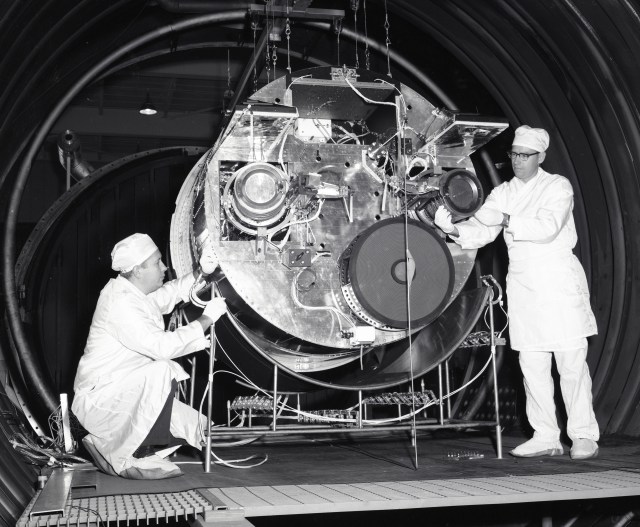
NASA’s SERT II: ‘A Genuine Space Success Story’
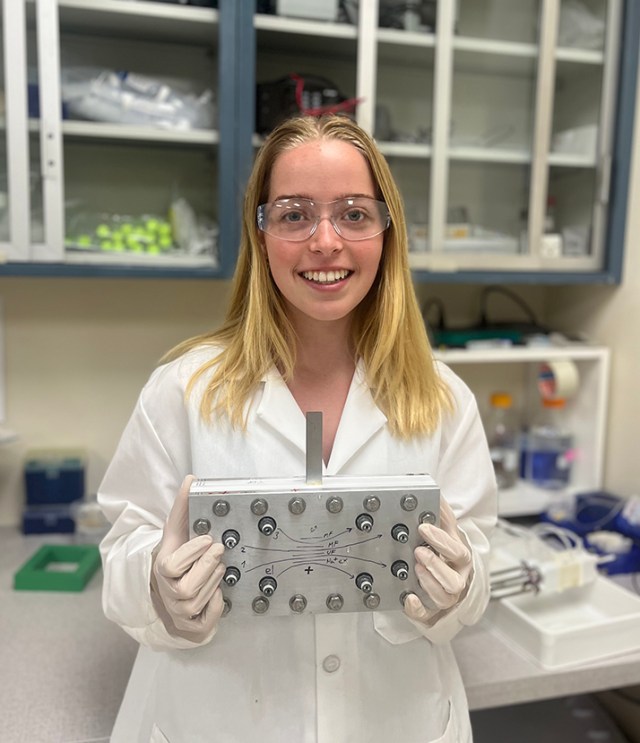
Tech Today: Synthetic DNA Diagnoses COVID, Cancer
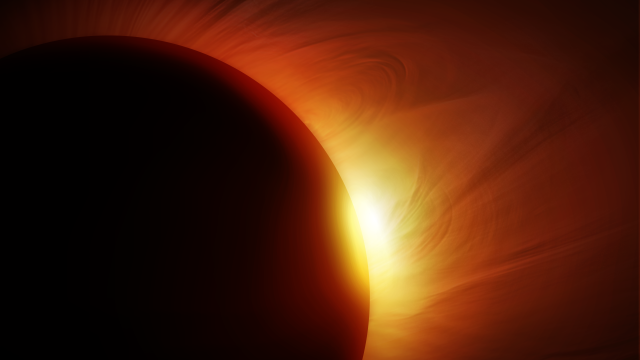
NASA Partnerships Bring 2024 Total Solar Eclipse to Everyone
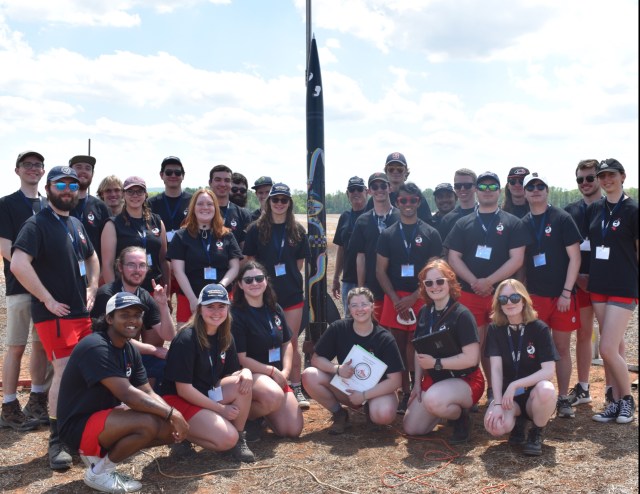
Launch Week Event Details
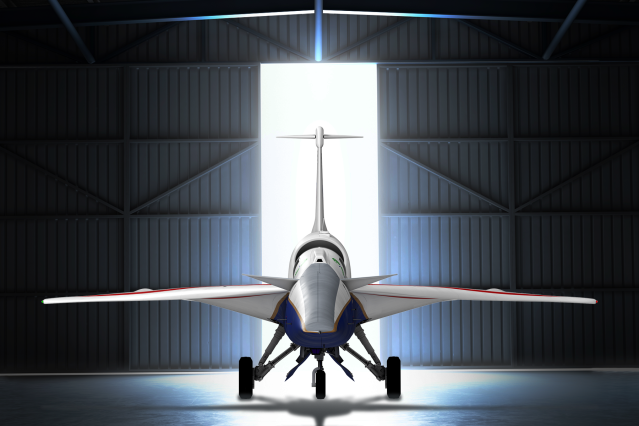
La presentación del X-59 de la NASA personifica la tradición aeronáutica
A researcher’s guide to: plant science, space station research integration office.
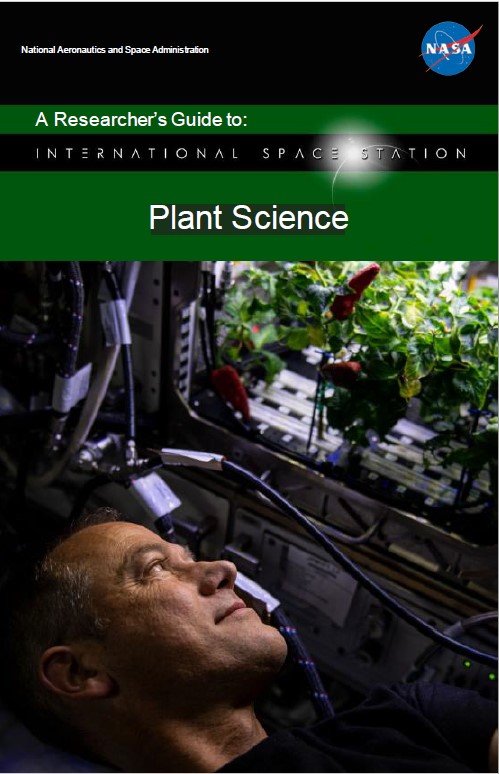
March 2023 Edition
By Elison B. Blancaflor, Ph.D. Raymond M. Wheeler, Ph.D. Gioia Massa, Ph.D Jeff T. Richards, M.S. Charles D. Quincy, PE Howard G. Levine, Ph.D.
Advances made during decades of spaceflight experimentation have identified critical gaps in our understanding of the role of gravity and the spaceflight environment on plant biology at the cellular, tissue, whole plant, and community levels.
The International Space Station is a unique platform where reduced gravity can be used to probe and dissect biological mechanisms in plants for understanding how terrestrial biology responds to gravity. This knowledge is important for supporting safe and long-term human habitation in space using bioregenerative life support with plants and microbial communities in space. This knowledge can also be applied to reducing exploration risks to crews by designing countermeasures to problems associated with living in space. In addition, scientists can investigate how plants respond to reduced gravity environments on the moon and Mars.
This researcher’s guide provides information on the background for plant science research, summarizes previous research, including lessons learned, and provides information on ISS research facilities. Information on what potential investigators should know about conducting research on the International Space Station is also provided.
PDF readers: PDF [1.6 MB]
All other eBook readers: ( in process )
Discover More Topics
Station Researcher’s Guide Series
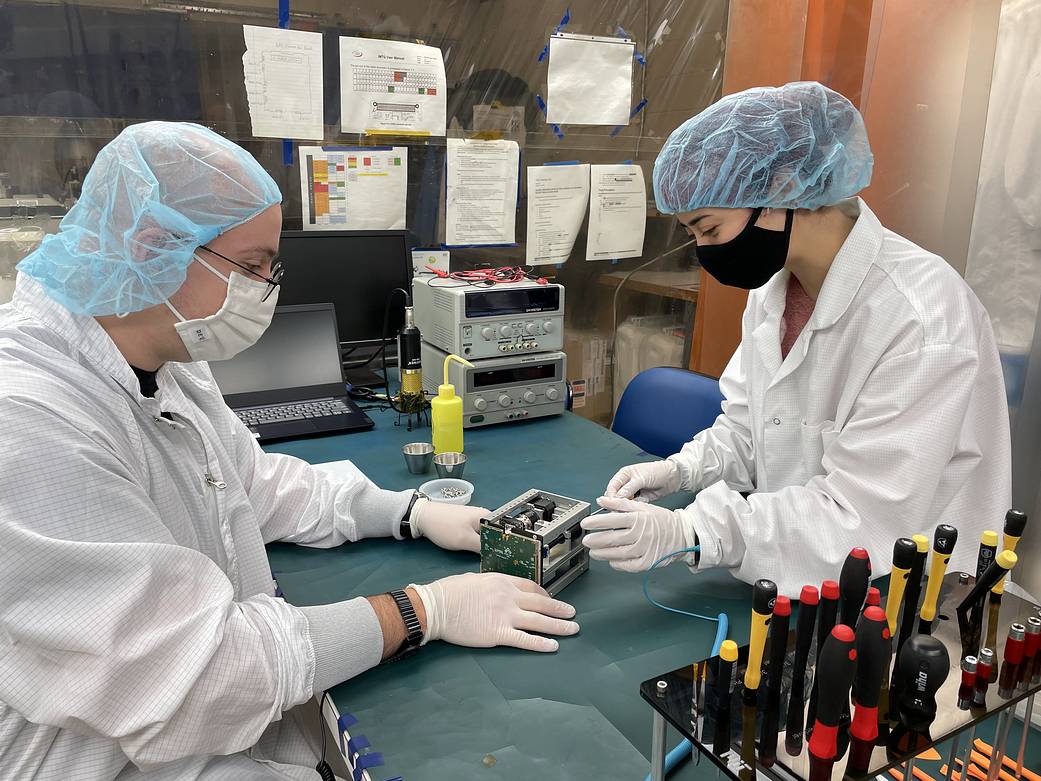
Opportunities and Information for Researchers
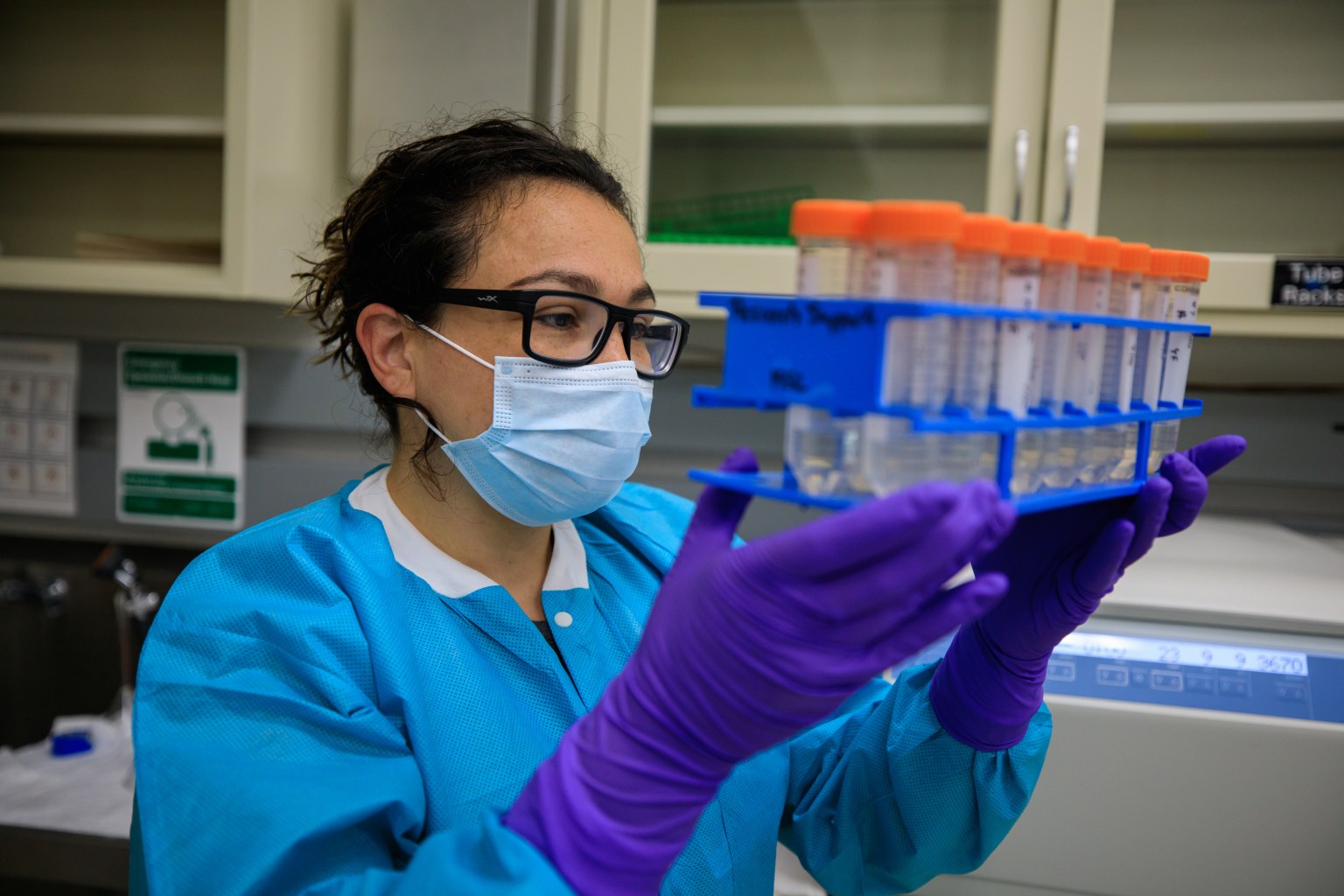
Space Station Research Results
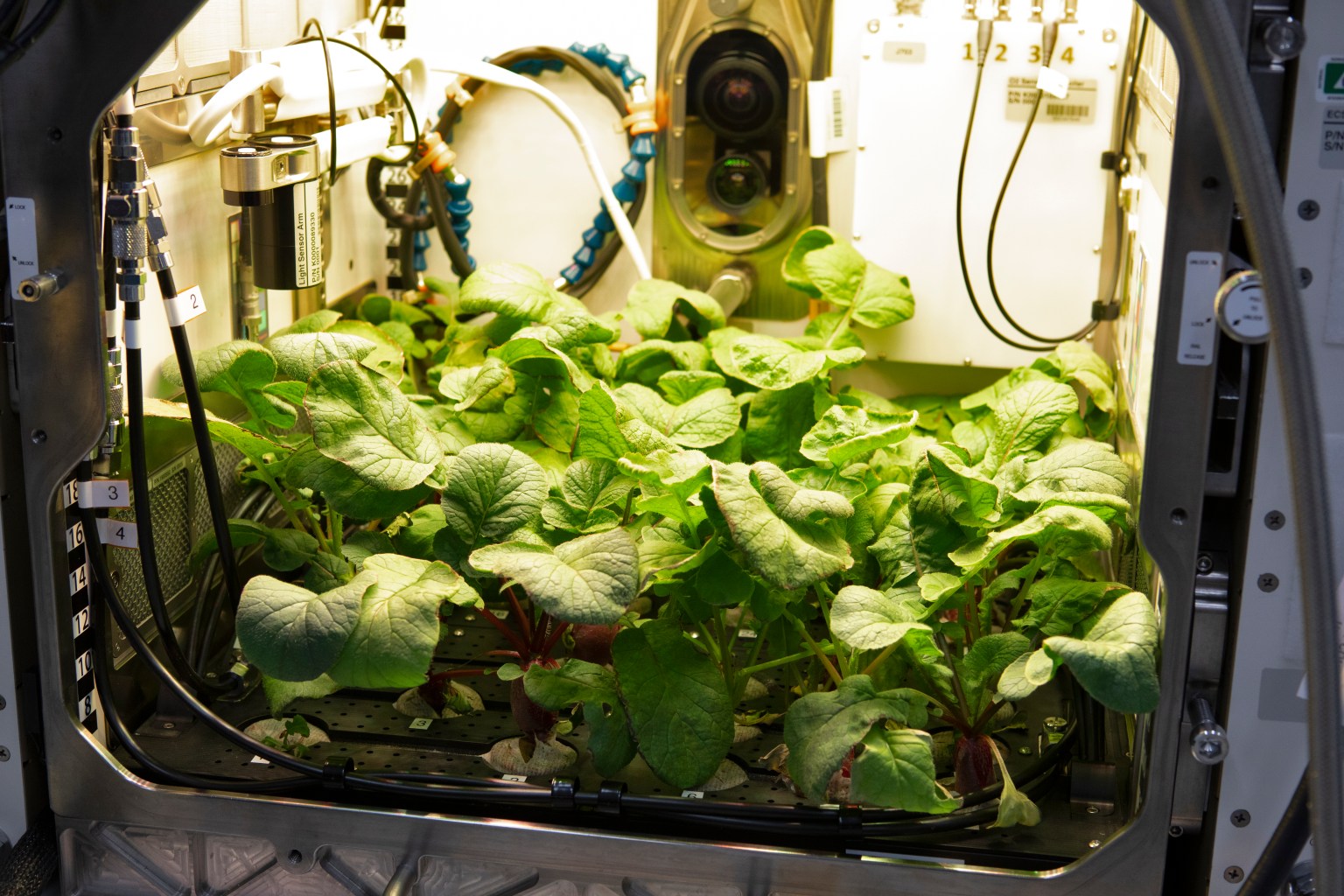
Latest News from Space Station Research
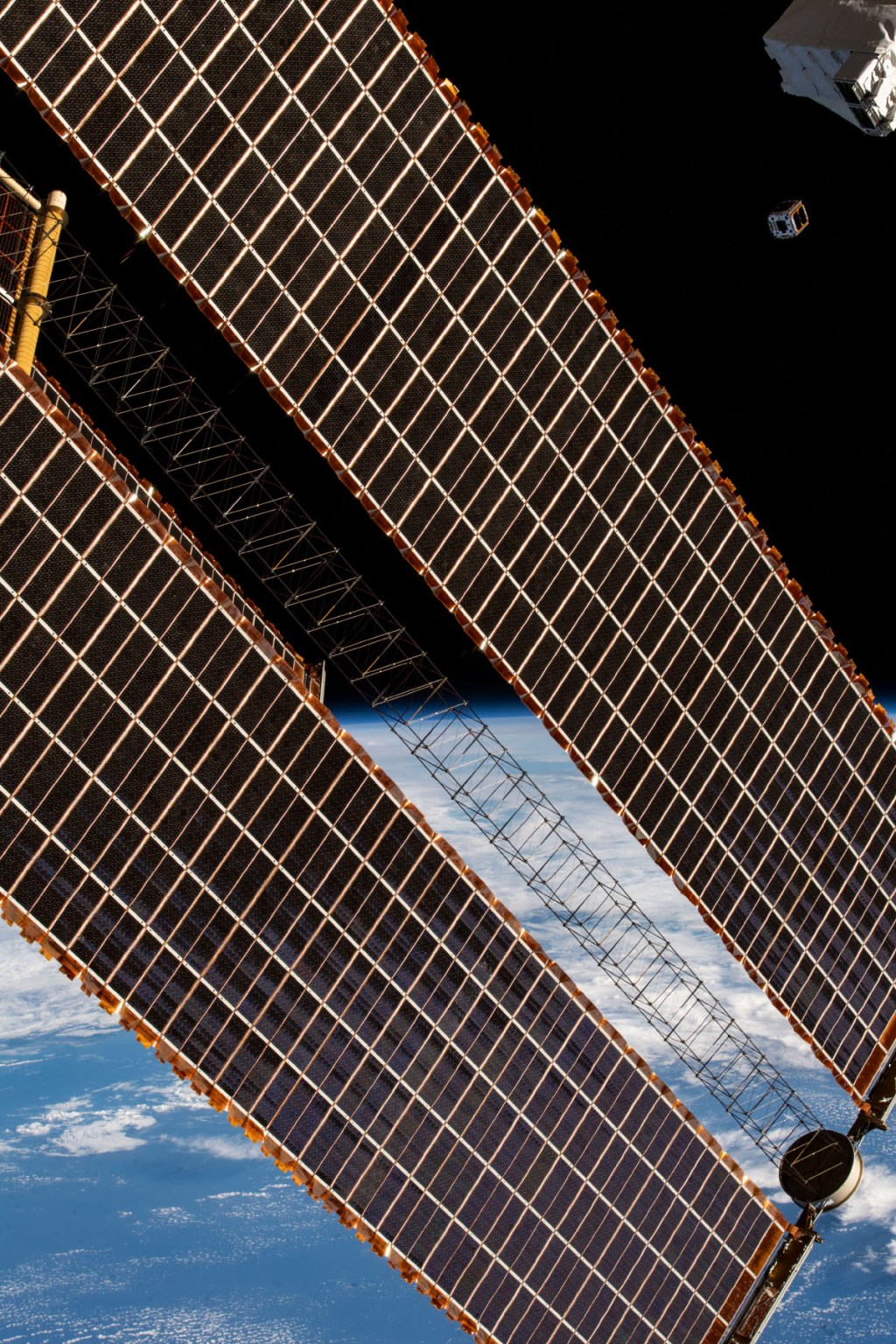

Smithsonian Environmental Research Center
Understanding ecosystems for a sustainable future, search form.
Visitors: The SERC campus is open Monday-Saturday, 8:00am-5:30pm. We're closed Sundays and federal holidays. Please do a health self-check before arriving, and stay home if feeling sick. Read Plan Your Visit for information on where to park, updated maps and hours, safety, and more.
Our trails are closed due to downed debris from a recent storm. For your safety, please do not hike the trails until further notice. We appreciate your patience while we work to clear the debris.
In the event of a government shutdown, SERC will remain OPEN for our normal Monday - Saturday hours through at least Saturday, October 7, by using prior year funds. Visit si.edu for updates.
The Woodlawn History Center is open Friday and Saturday, 10:00am - 2:00pm. Read Plan Your Visit for information on where to park, updated maps, safety, and more.
- Strategic Goals
- Advisory Board
- Corporate Leaders Program
- Director's Letter
- SERC Newsletter
- SERC Newsletter Signup
- Research Topics
- Laboratories
- Publications
- Research Around the Globe
- Coastal Carbon Network
- Ecological Genomics Core
- Technology in Ecology
- Environmental Data
- National Ballast Information Clearinghouse
- On-site School Programs
- On-site Group Programs
- Volunteer in Education
- Professional Development & Science Courses
- Learning Resources
- Virtual Field Trips & Talks
- Job Opportunities
- Visiting Scientists and Research Associates
- Internships and Fellowships
- Plan Your Visit
- Calendar of Events
- Maps and Trails
- Evening Lectures
- Woodlawn History Center
- SERC Fact Sheet
- Find an Expert
- Image Gallery
- News Releases
- SERC In The News
- Smithsonian Statement on Responding to Climate Change
- Science Writing Internships
- Why Do We Call It Participatory Science?
- Volunteer Projects
- Annual Reports
- Sign Up For The Email List
- Shorelines Blog
- SERC YouTube Channel
- Meeting Spaces & Housing
Plant Growth
Education Menu
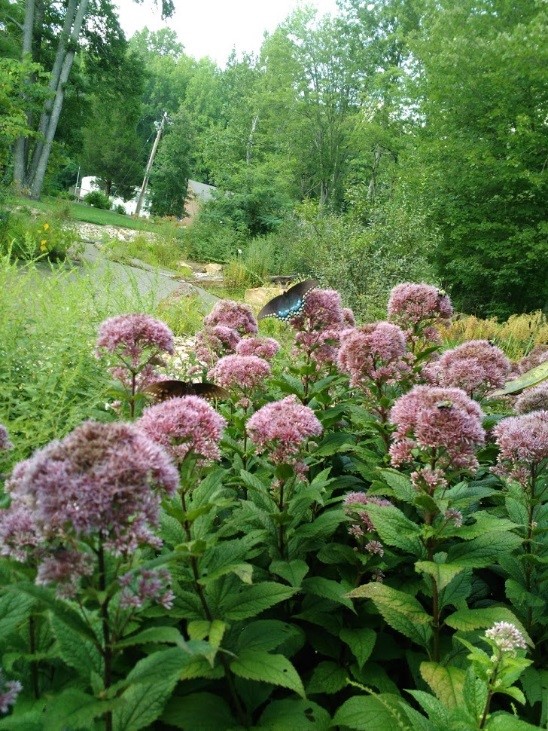
Plants are vital to all life on Earth. They are important because plants take in carbon dioxide from the atmosphere and produce oxygen. In addition, plants make up the base of the food web by producing their own food using light, water, carbon dioxide, and other chemicals. This is why they are known as producers or Autotrophs . Examples of autotrophs on land are trees, vines, or shrubs. Autotrophs can also be found in the upper layers of the ocean, called algae. One of the many services autotrophs provide is to protect against erosion. Erosion is when the force of water, wind, or ice wash away layers of the soil that are necessary to protect against strong weather events like thunderstorms or hurricanes. If you lived on a hill that had little or no autotrophs and it rained really hard, over time, you would be at risk for landslides because their roots hold the soil together. Luckily, there are people who study plants, called botanists or plant ecologists/ scientists, and can tell us about why plants are needed! These scientists study the biology and chemistry of plants. In other words, they study the function of plants and why plants evolved the way they did, among other things. SERC has plant ecologists that study autotrophs and their interactions with other autotrophs, animals, and other living organisms. Read more about their research here: Plant Ecology at SERC .
- Middle School
- Germination is when the seed has become a tiny plant.
- To sow seeds means to plant or scatter them in soil so they can start growing.
- Photosynthesis is a chemical process that explains the way plants eat. It is also one of the processes that produce oxygen.
- Frost date is the predicted day when it will be too cold to plant anything. This date is important to know because if it is too cold, your new plants will not do well so you have to wait until after this date to plant.
- Plant hardiness zone helps growers determine what plants can thrive at a specific location. Plants will do well in the zone they are labeled at and in the zone numbers higher than that. For example, a plant that is hardy at zone 5 should be able to survive in zones 5-13 ( Zoning in on Hardiness , What zone am I in? ).
- Temperature is a measurement of how hot or cold something is. It is measured in Fahrenheit (F) and Celsius (C) degrees . Temperature in Celsius is commonly used in science, but it is really up to your teachers and school to tell you if they prefer Fahrenheit or Celsius.
- Pollination is the transfer of pollen from one plant to another. This happens when pollen sticks to a pollinator such as a bee, bird, or bat while feeding on the nectar of a flower.
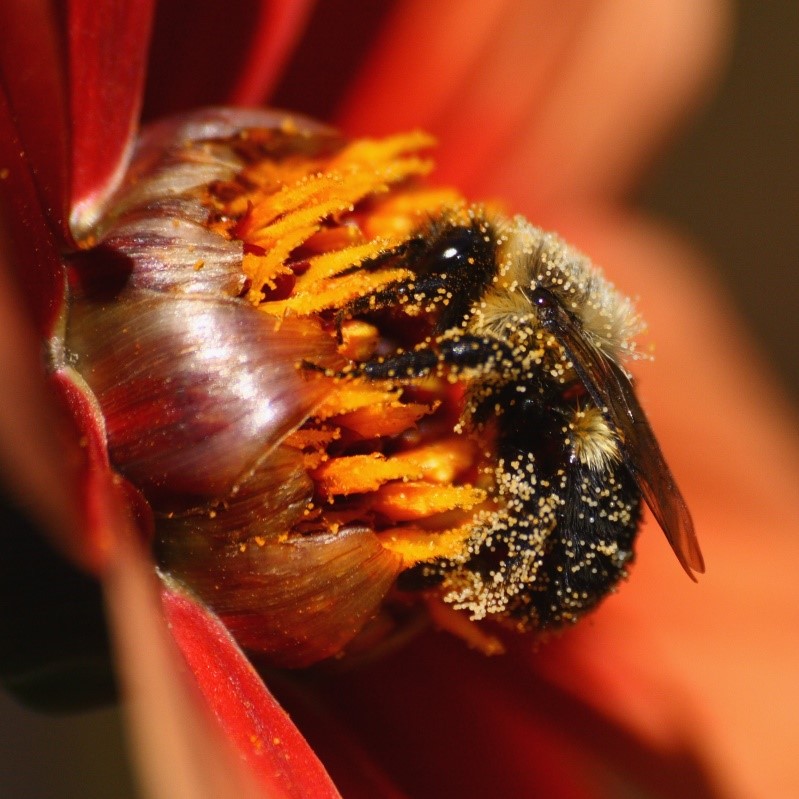
- Greenhouse gases , as stated by the Environmental Protection Agency (EPA) , are gases such as carbon dioxide and methane that trap heat in the Earth’s atmosphere. These greenhouse gases create the Greenhouse Effect which warms Earth’s surface (where we live) so we can live at a comfortable temperature.
- NASA - What are climate and climate change? (for students in grades 5-8)
- NASA's Climate Kids - Global Climate Change
- Watch this video to learn more about the causes and effects of climate change: National Geographic - Causes and Effects of Climate Change video
- Read more about the Greenhouse Effect by going to this link: NASA Climate Kids - What is the greenhouse effect?
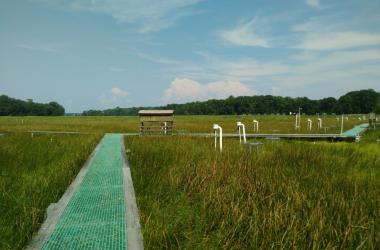
Connections to SERC's Research About Plant Growth
Photosynthesis, Nutrients, Soil & Basic Plant Information
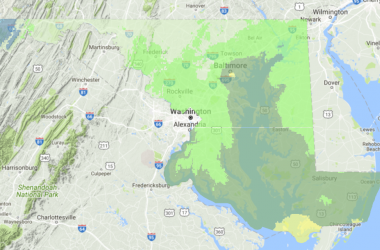
What You Need to Know About the Location You Are Planting In
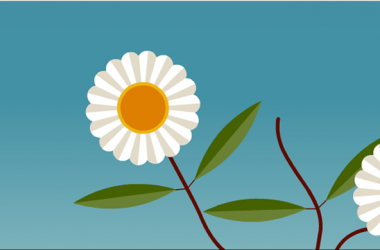
Extinct- Plant Survival Game
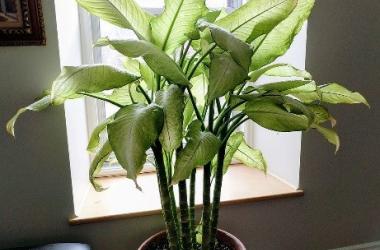
How to Grow A Plant Indoors/ Outdoors Plus Supplies
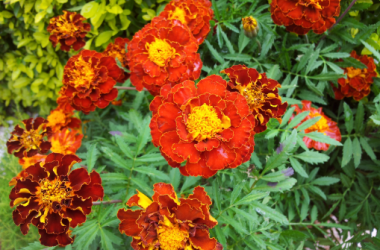
Examples of Plants You Can Grow for Every Season
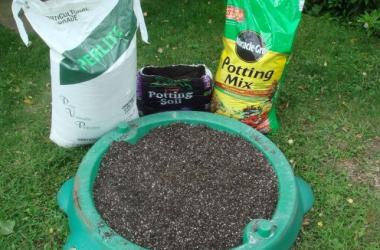
Types of Soil for Gardening

- News Releases
An inside look at how plants and mycorrhizal fungi cooperate
A new view into a symbiosis could offer benefits to address climate change: pulling atmospheric carbon into soil and boosting biofuel feedstocks with less fertilizer
DOE/Lawrence Berkeley National Laboratory
Benjamin Cole, senior author of the Nature Plants paper describing one of the first cross-kingdom spatially-resolved transcriptomics studies to date.
Credit: (Thor Swift/Berkeley Lab)
For millions of years, underground fungi have lived in symbiosis with plant roots. Plants provide photosynthesized carbon, while fungi deliver water and nutrients. In order to do so, these organisms share space at cellular scale: fungi stretch a network of tendrils called arbuscules into a plant’s root cells, and both organisms rearrange their cells around this structure to facilitate sharing.
Recently, researchers have been able to study both sides of this interaction up close, using RNA sequencing to understand gene expression: one of the first cross-kingdom spatially-resolved transcriptomics studies to date. This paper is the cover article for the April 2024 issue of Nature Plants .
“We wanted to better understand the nature of this symbiosis at the cellular level — really understand how those two cell types [of two different organisms] are interacting with each other, without all the noise or other biological activity of the surrounding neighborhood,” said Benjamin Cole, senior author of this work. Cole is a research scientist at the U.S. Department of Energy (DOE) Joint Genome Institute (JGI), a DOE Office of Science User Facility located at Lawrence Berkeley National Laboratory (Berkeley Lab).
Specific understanding of this symbiosis could offer improvements in multiple directions. On the fungi side, underground mycorrhizal networks can serve as a reservoir for carbon compounds that plants generate from carbon dioxide they take in. In this way, encouraging this symbiosis could improve the way soils store carbon from the atmosphere. For plants, boosting this relationship could improve biofuel feedstocks growing in nutrient-poor fields. “This is one interaction that allows plants to better survive in those environments,” Karen Serrano said. She is first author of this paper and a graduate researcher at the Joint BioEnergy Institute (JBEI).
Cole led the work in collaboration with JBEI as part of the 2021 Laboratory Directed Research and Development (LDRD) program. Cole also received a DOE Early Career Research Program (ECRP) award in 2021, which aims in part to build off of this work. Former JGI postdoctoral scholar Margot Bezrutczyk collaborated extensively with Cole and Serrano to collect and analyze the single nuclei and spatial transcriptomics data this work leverages.
These experiments focused on two species at once: the model legume species Medicago truncatula and the mycorrhizal fungi Rhizophagus irregularis. To see how these organisms cooperate, this team applied R. irregularis spores directly to M. truncatula seedlings grown in a controlled environment chamber, so the fungus could colonize the plants’ roots. Then, comparing with control seedlings which were not treated with fungi, they used multiple approaches to look at gene expression in both plant cells and fungal cells.
Using a technique called single nuclei RNA sequencing, the researchers identified different cell types within M. truncatula root cells, and profiled their gene expression. Then, the researchers used a technique called spatial transcriptomics, to generate maps of gene expression. This spatial transcriptomics technique allowed them to understand gene expression within circular capture areas roughly 55-microns in diameter — about the width of a human hair. At such resolution, this spatial transcriptomics data captured molecular information from both plant cells and fungal cells.
“Because this technology relies on just polyadenylated transcript capture — any RNA that is from eukaryotes — we were able to capture both plant and fungal transcripts,” Serrano said. This team quantified the expression of over 12,000 fungal genes, in addition to associated plant genes.
All together, these data offer a granular view of both plant and fungal activity at different stages of this symbiosis. Within that activity, Serrano, Cole and their team found over 1,000 upregulated genes, 188 of which were shared with previous studies in the same system. With the right functional characterization, those genes could become dials for tuning this symbiosis. “Those are great candidates for genetic engineering. Our hope is that the community at large will follow that up,” Serrano said.
This work focused on a relatively well-understood model system, so future directions will also include targeting biofuel feedstocks in similar studies. “We would like to look at arbuscular mycorrhizal symbiosis in other bioenergy grasses, like sorghum and switchgrass. We're optimizing systems now, so that we can get those working,” Cole said.
The U.S. Department of Energy Joint Genome Institute, a DOE Office of Science User Facility at Lawrence Berkeley National Laboratory, is committed to advancing genomics in support of DOE missions related to clean energy generation and environmental characterization and cleanup. JGI provides integrated high-throughput sequencing and computational analysis that enable systems-based scientific approaches to these challenges. Follow @jgi on Twitter.
DOE’s Office of Science is the largest supporter of basic research in the physical sciences in the United States, and is working to address some of the most pressing challenges of our time. For more information, please visit science.energy.gov .
The Joint BioEnergy Institute is a DOE Bioenergy Research Center managed by Berkeley Lab. For more information, please visit jbei.org .
Nature Plants
10.1038/s41477-024-01666-3
Method of Research
Experimental study
Subject of Research
Not applicable
Article Title
Spatial co-transcriptomics reveals discrete stages of the arbuscular mycorrhizal symbiosis
Article Publication Date
Disclaimer: AAAS and EurekAlert! are not responsible for the accuracy of news releases posted to EurekAlert! by contributing institutions or for the use of any information through the EurekAlert system.
Additional Multimedia
Original Source

- Search this journal
- Search all journals
Advertisement
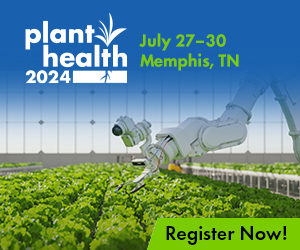
Article Sections
- Materials and Methods
- Results and Discussion
Article Figures
Vegetative top dry weight (DW), leaf DW, stem DW, and stem diameter of mature eggplant plants as affected by irrigation rate. Irrigation rate was applied as percentage of crop evapotranspiration. Curve was fit by linear regression. Fall of 2010, Tifton, GA.
Seasonal volumetric soil water content (measured at 12- and 30-cm depth) as influenced by irrigation rate. Irrigation rate was applied as percentage of crop evapotranspiration. Line was fit by linear regression. Fall of 2010, Tifton, GA.
Effect of irrigation rate and soil depth on the concentration of nitrate-nitrogen in the soil (0 to 60 cm) in drip-irrigated eggplant grown on raised beds and plastic film mulch. Irrigation rate was applied as percentage of crop evapotranspiration. Line was fit by linear regression. Fall of 2010, Tifton, GA.
Cumulative number of fruit and fruit yields as affected by irrigation rate in drip-irrigated eggplant grown on raised beds and plastic film mulch. Irrigation rate was applied as percentage of crop evapotranspiration. Line was fit by linear regression. Fall of 2010, Tifton, GA.
Individual fruit weight as influenced by irrigation rate in drip-irrigated eggplant grown on raised beds and plastic film mulch. Irrigation rate was applied as percentage of crop evapotranspiration. Line was fit by linear regression. Fall of 2010, Tifton, GA.
- View raw image
- Download Powerpoint Slide
Related Content
- Previous Article
- Next Article

Eggplant ( Solanum melongena L.) Plant Growth and Fruit Yield as Affected by Drip Irrigation Rate
Eggplant ( Solanum melongena L.) is an increasingly popular crop in the United States. In the southeastern United States, eggplant is often produced with high levels of irrigation water [above the rate of crop evapotranspiration (ETc)], resulting in water waste and nitrogen (N) leaching. The objective of this research was to assess the effects of irrigation rate on plant growth and fruit yield in eggplant. The study was conducted in Tifton, GA, in the fall of 2010 and 2011. Eggplant plants cv. Santana were grown on raised beds (1.8 m centers) covered with white plastic film mulch. There was a single drip tape along the center of the bed. The design was a randomized complete block with five treatments and four replications. Treatments consisted of irrigation rates based on ETc (33%, 67%, 100%, 133%, and 167% ETc). Plant growth, chlorophyll index (CI), and volumetric soil water content (SWC) were monitored over the season. In 2010, SWC (0–30 cm deep) increased and soil nitrate levels decreased with increasing irrigation rates. Foliar N and potassium (K), and CI decreased with increasing irrigation rate, probably due to a dilution effect. Stem diameter, leaf dry weight (DW), and vegetative top DW increased with increasing irrigation rate. Net photosynthesis and stomatal conductance ( g S ) were lowest at 33% ETc. Fruit number and fruit yields (marketable and total) were also lowest at 33% ETc and there were little yield differences among irrigation rates higher than 33% ETc. In 2011, irrigation rate had minor or no effect on SWC, plant growth of mature plants, leaf gas exchange, and fruit number and yield. The no treatment effect observed for eggplant in 2011 was likely because study was conducted in a low field that remained moist most of the time, nullifying the treatment effects. Results suggested that eggplant may tolerate mild water stress, since plants irrigated at 67% ETc produced fruit yields similar to those of plants irrigated at 100% ETc or higher rates. Thus, there is a potential to save water by reducing current irrigation rates without negatively impacting fruit yields.
Eggplant, also known as aubergine and brinjal, is widely grown and consumed in southern and southeast Asia and has increased in popularity in the United States as a specialty vegetable. In 2001, U.S. eggplant production was valued at $42.5 million, and Georgia, Florida, California, New Jersey, and New York were the top five producers. The U.S. Department of Agriculture has not collected complete domestic production statistics for eggplants since 2001. In 2012, farm gate value in the state of Georgia was $17 million ( CAED, 2013 ). Average eggplant yield in Florida is ≈30 t·ha −1 ( Ozores-Hampton, 2014 ).
Eggplant is in the Solanaceae family, as are tomato ( Solanum lycopersicon ) and pepper ( Capsicum annum ) and shares similar environmental and cultural requirements as those crops. However, in contrast to tomato and pepper, eggplant crop can tolerate greater levels of drought stress ( Behboudian, 1977 ). There are several studies on eggplant irrigation carried out in Asia, Africa, and Europe ( Aujla et al., 2007 ; Behboudian, 1977 ; Chartzoulakis and Drosos, 1995 ; Gaveh et al., 2011 ; Karam et al., 2011 ) showing that eggplant can be produced at moderate levels of drought stress without major impact on fruit yield.
In southeastern United States, eggplant is often produced with high levels of irrigation water (above the rate of ETc) and N fertilizer, resulting in water waste and N leaching. Excessive irrigation rate not only wastes water, but may also result in reduced yields in bell pepper ( Díaz-Pérez et al., 2004 ; Sezen et al., 2006 ) and tomato ( Locascio et al., 1989 ; Ngouajio et al., 2007 ). To our knowledge, there are no published studies in the United States on the effect of irrigation rate on the yield and plant growth of drip-irrigated eggplants. Irrigation studies, intended to optimize use of irrigation water, are necessary to enable the protection of water resources in the United States. Therefore, the objective of this research was to assess the effects of irrigation rate on plant growth and fruit yield in eggplant.
Study site.
The study was carried out at the Horticulture Farm, University of Georgia, Tifton, GA, during the fall of 2010 and 2011. The farm is located at an altitude of 108 m above mean sea level, 31°28′ N latitude and 83°31′ W longitude. The soil of the farm is a Tifton sandy loam (a fine loamy-siliceous, thermic Plinthic Kandiudults) with pH 6.5. Available water capacity is 18 to 36 mm in the top 30 cm of soil profile ( Calhoun, 1983 ). In 2010, field had a gentle sloping (slope ≈3%); in 2011, field had a nearly level slope. The distance between the 2010 and 2011 fields was ≈70 m.
Land preparation and planting.
Eggplant plants were grown on plastic film mulch on raised beds (6 × 0.76 m, formed on 1.8-m centers). Before laying mulch, the soil was fertilized with N, phosphorous (P), and K at 60, 26, and 50 kg·ha −1 , respectively, using 10–10–10 granular fertilizer. At the same time, plastic film mulch [white on black, low-density polyethylene with a slick surface texture, 1.52 m wide and 25 µm thick (RepelGro, ReflecTek Foils, Inc., Lake Zurich, IL)] was laid with a mulch-laying machine, drip irrigation tape [20.3 cm emitter spacing and a 8.3 mL·min −1 emitter flow (Ro-Drip, Roberts Irrigation Products, Inc., San Marcos, CA)] was placed 5 cm deep in the center of the bed.
Eggplant transplants were produced in a greenhouse using peat-based medium (Pro-Mix, Quakertown, PA) and polystyrene 200-cell (2.5 × 2.5 cm cell) trays. Six-week-old eggplant transplants were planted with a mechanical transplanter on 6 Aug. 2010 and 5 Aug. 2011 in one row per bed, with a 60 cm separation between plants. About 250 mL of starter fertilizer solution (555 mg·L −1 N; 821·mg·L −1 P; 0 mg·L −1 K) was applied directly to the base of each transplant. The length of the experimental plot was 6.1 m. Starting 3 weeks after transplanting, plants were fertilized weekly through the drip system with N and K. Fertilization rates of N and K after transplanting were 0.7, 1.0, 1.5, and 2 kg·ha −1 ·d −1 in week 5, week 6; week 7; and weeks 13–15, respectively. Total N–P–K applied in the season was 218 kg·ha −1 N, 30 kg·ha −1 P, and 181 kg·ha −1 K.
Experimental design and treatments.
The design was a randomized complete block with five treatments and four replications. Treatments consisted of irrigation rates based on ETc (33%, 67%, 100%, 133%, and 167% the rate of ETc). ETc was calculated by multiplying the reference evapotranspiration (ETo) by a crop coefficient (Kc), which is dependent on the crop stage of development. Available Kc values for eggplant were developed for bare soil (unmulched) production. These Kc values, however, are not recommended for crops under plasticulture systems since plastic mulches reduce soil evaporation and ETc ( Allen et al., 1998 ; Pereira et al., 2015 ; Simonne et al., 2006 ). The Kc values used in this study were modified relative to those proposed for bell pepper in Florida ( Simonne et al., 2006 ). The Kc values used were 0.25 (week 1 after transplanting), 0.40 (week 2), 0.55 (week 3), 0.70 (week 4), 0.85 (week 5), 1.0 (week 6–11), and 0.8 (week 12–14).
All treatments received equal volumes of irrigation water (88 and 49 mm in 2010 and 2011, respectively) during the crop establishment period (first 4 weeks after transplanting). Irrigation treatments were initiated on week 5. Water was applied when cumulative ETc was ≈12 mm, which corresponded to about every 2 to 3 d in mature plants (mean ETo was 5 to 6 mm·d −1 ). Thus, amounts of water per irrigation event were ≈4 mm (33% ETc), 8 mm (67% ETc), 12 mm (100% ETc), 16 mm (133% ETc), and 20 mm (167% ETc).
Soil water content.
Soil water content (volumetric) in the 0–12 cm of soil profile over the season was measured manually once every 2–3 d (three readings per experimental plot) with a portable time-domain reflectometry (TDR) sensor (CS-620; Campbell Scientific, Logan, UT). The two metallic 12-cm rods of the TDR sensor were inserted vertically within the row between two plants. Soil water content (volumetric) in the 0–30 cm of soil profile was periodically (every 10 min) monitored with TDR sensors (CS-610; Campbell Scientific) connected to a datalogger (CR-10X; Campbell Scientific). The moisture sensors had three metallic 30-cm rods and were inserted vertically within the row between two plants.
Soil nitrate.
Soil samples were taken from each plot at 0- to 20-cm, 20- to 40-cm, and 40- to 60-cm depths on 8 Nov. 2010. Samples were taken at least 0.5 m away from the borders of the plots and from the previous sampling holes. Samples were air-dried and analyzed for nitrate-nitrogen using standard QuickChem Methods (Lachat Quick-Chem 8000 FIA; Zellweger Analytics, Milwaukee, WI).
Plant growth.
Eggplant plant height and stem diameter were measured weekly in three mature plants per plot. Plant samples obtained at the end of the season were dried at 70 °C for several days until constant weight was obtained. Leaf, stem, and vegetative top (leaf + stem) DW of individual plants were determined.
Chlorophyll indices were determined twice a week over the season on six mature, well-exposed, and healthy leaves per plot using a chlorophyll meter (Chlorophyll Meter SPAD-502; Minolta Co., Ltd., Ramsey, NJ).
Leaf gas exchange and PSII efficiency.
Simultaneous measurements of leaf gas exchange (net photosynthesis, g S , transpiration, and internal CO 2 concentration), and fluorescence were determined as PSII efficiency were made with an infrared gas analyzer (LI-COR 6400 IRGA with an integrated 6400-40 leaf chamber fluorometer; LI-COR, Inc., Lincoln, NE). PSII efficiency is the fraction of absorbed PSII photons used in photochemistry and is measured with a light-adapted leaf. Water use efficiency (WUE) was calculated as the ratio between leaf net photosynthesis and leaf transpiration. Air flow rate was set at 300 µmol·m −2 ·s −1 on the reference side. The CO 2 concentration was set at 400 µmol·mol −1 with a CO 2 mixer and a CO 2 tank. Measurements were conducted in developed plants on clear days (photosynthetically active radiation ≈2000 µmol·m −2 ·s −1 ) at 1200–1500 hr Eastern Standard Time in 2010 (6 and 20 Oct. and 9 Nov.) and 2011 (5 Oct.), using two developed and fully exposed leaves per experimental plot.
Leaf mineral nutrients.
Leaf samples (20 fully developed leaves from new growth) from developed plants were dried at 70 °C for 2 d and analyzed for mineral nutrient concentration at the University of Georgia, Agricultural & Environmental Services Laboratories, Athens, GA.
Weather data (air temperature, ETo, and rainfall) were obtained from a nearby University of Georgia weather station (within 300 m).
The harvest lasted from 28 Sept. to 23 Nov. in 2010 and from 23 Sept. to 4 Nov. in 2011. Eggplant fruit were harvested twice per week at commercial stage. Harvested section consisted of 10 plants per plot. Fruit were graded according to U.S. Department of Agriculture standards ( USDA, 2013 ) as marketable or cull and number and weight of marketable and cull fruit were determined. Average fruit weight was derived mathematically from the total weight and the total number of fruits.
Irrigation water use efficiency.
Irrigation water use efficiency (IWUE) was calculated by dividing fruit weight (kg·ha −1 ) by irrigation water received by the crop (in mm) for each irrigation treatment.
Agronomic efficiency of nitrogen.
Agronomic efficiency of nitrogen was calculated by dividing total eggplant fresh fruit weight (kg·ha −1 ) by the amount of N (kg·ha −1 ) applied to the crop.
Fruit DW content and harvest index (HI).

Statistical analysis.
Data were analyzed using the General Linear Model and Regression Procedures from SAS (SAS version 9.3, SAS Institute Inc., Cary, NC). Data means were separated by Fisher’s protected least significant difference test at 95% confidence and response curves determined by orthogonal contrasts. Percentages were transformed to arcsin values before analysis. For clarity, nontransformed percentage means were used for presentation in tables and figures. Data from all years were pooled if no year × treatment interactions were found.
In 2010, average maximal, mean, and average minimum air temperature for the season were 28.8, 22.6, and 16.4 °C, respectively. Cumulative ETo and rainfall for the season were 370 and 184 mm, respectively. In 2011, average maximal, mean, and average minimum air temperature were 28.6, 22.5, and 16.4 °C, respectively. Cumulative ETo and rainfall for the season were 344 and 256 mm, respectively.
In 2010, vegetative top DW, leaf DW, stem DW, and stem diameter increased with increasing irrigation rate ( Fig. 1 ). Leaf weight ratio (LWR) [leaf biomass as a fraction of vegetative aboveground biomass (mean = 0.529)] decreased with increasing irrigation rate ( r 2 = 0.92; P ≤ 0.05) from LWR of 0.543 at 33% ETc to LWR of 0.493 at 167% ETc, which indicates that plants allocated less biomass to leaves as irrigation rate increased. Bell pepper leaves have reduced leaf thickness at low light and low water stress conditions ( Díaz-Pérez, 2013 ). In 2011, over the season, mean stem diameter was lowest at 33% ETc ( P < 0.05), although final stem diameter was unaffected by irrigation rate ( Table 1 ). Mean seasonal plant height increased with irrigation rate, ranging from 66 cm (33% ETc) to 93 cm (167% ETc); final plant height (4 Nov.) was unaffected by irrigation rate. Mature plant DW (mean = 1.70 kg) was also unaffected by irrigation rate. Growth differences during midseason but not at the end of the season were probably because of high evaporative demand conditions that impacted plant growth at low irrigation rates during midseason. Late in the season, when evaporative demand was reduced, the effect of irrigation rate on plant growth was less detectable. The no treatment effect observed for eggplant in 2011 was likely because study was conducted in a low field that remained moist most of the time, nullifying the treatment effects.

Citation: HortScience horts 50, 11; 10.21273/HORTSCI.50.11.1709
- Download Figure
Plant growth, leaf chlorophyll index (CI), and soil water content (SWC) as affected by irrigation rate in eggplant. Fall of 2011, Tifton, GA.

Reduced eggplant plant growth at irrigation rates below 100% ETo has been previously reported. Eggplant irrigated at 80% pan evaporation, every 8 d, and 70% pan evaporation, every 12 d, had reduction of 18% and 27% in plant height, and 13% and 21% in stem diameter, respectively ( Kirnak et al., 2002 ). In bell pepper exposed to different soil water levels by varying drip emitter spacing, plant height and canopy diameter increased with decreasing emitter spacing (i.e., with increased soil water levels) ( Madramootoo and Rigby, 1991 ).
In 2010, CIs decreased with increased irrigation rate ( P = 0.006), from 60.8 at 33% ETc to 59.0 at 167% ETc. In 2011, CI decreased from 55.8 at 33% ETc to 53.7 at 167% ETc ( Table 1 ). Decreased CI values with increased irrigation rates were likely due to dilution effect of nutrients, since plant growth was enhanced with increased irrigation rates. Decreased CI with increased irrigation rates may also be associated with increased nitrate leaching under high irrigation rates.
In 2010, the effect of irrigation rate on SWC varied with soil depth. At 0- to 30-cm depth, SWC increased with increasing irrigation rates ( Fig. 2 ), whereas at 0- to 12-cm depth SWC was unaffected by irrigation rate. Differences in soil moisture in the different soil depths indicate a higher soil water uptake by plants, because of greater presence of roots at 0–12 cm than at 0- to 30-cm depth; they also indicate that high rates of irrigation (>100% ETc) result in wasted water because much water at 0- to 30-cm depth was not taken up by the crop; and they suggest that soil moisture measurement at 0- to 30-cm depth was more sensitive to detect changes in soil moisture than measurement at 0- to 12-cm depth.

As in 2010, seasonal SWC at 0- to 12-cm depth was also similar among irrigation rates (mean = 13.4%) in 2011. In addition to the high presence of roots at 0- to 12-cm depth, SWC values were similar among treatments in 2011 probably because the study was conducted in a low field, with a nearly level slope, where soil was commonly moist throughout the season, likely due to lateral water movement from upper sections of the field. There was an impermeable clay layer 30- to 40-cm deep in the soil profile that probably allowed water to flow from upper to lower areas within the farm.
Leaf gas exchange.
In 2010, the effect of irrigation rate on leaf gas exchange varied by date ( Table 2 ). Net photosynthesis, g S , and photosynthetic WUE were unaffected by irrigation rate on 6 Oct. 2010. Lack of treatment differences on 6 Oct. was probably attributable to relatively low temperatures on day of measurement (mean temperature = 16.4 °C), resulting in low crop evaporative demand and low crop water stress. Net photosynthesis and g S were lowest at 33% ETc on 20 Oct. and 9 Nov. Water use efficiency was highest and PSII efficiency was lowest at 33% ETc on 20 Oct. The fact that gas exchange variables were not reduced at 67% ETc compared with higher irrigation rates suggests that plants at 67% ETc were likely unaffected by water stress. However, since gas exchange measurements were conducted only in mature plants, late in the season, when evaporative demand was reduced, it is possible that earlier in the season plants may have had experienced increased water stress at reduced irrigation rates, as suggested by the reduced plant growth at reduced irrigation rates. In 2011, leaf net photosynthesis (mean = 28.3 µmol·m −2 ·s −1 ), g S (mean = 0.248 mol·m −2 ·s −1 ), WUE (mean = 4.24 µmol·mmol −1 ), and PSII (mean = 0.189 µmol·mmol −1 ) were unaffected by irrigation rate. Air maximal and minimal temperature on the day of measurement were 27.5 and 11.0 °C, respectively. Lack of differences in gas exchange are consistent with the lack of differences in plant growth among irrigation rates observed in 2011.
Leaf gas exchange and fluorescence as affected by irrigation rate and date in eggplant. Fall of 2010, Tifton, GA.

Irrigation at 33% ETc was probably insufficient to satisfy eggplant water requirements, as suggested by the reduced leaf gas exchange values ( Table 2 ). Reduced irrigation rates can result in decreased gas exchange in solanaceous crops. Transpiration, leaf g S , and leaf net photosynthesis in eggplant were reduced with water stress and effects varied depending on stress severity and duration ( Sarker et al., 2005 ). In habanero pepper ( Capsicum chinense Jacq.), there was reduced g S and net photosynthesis with increased time between irrigations ( Jaimez et al., 1999 ).
Soil nitrate concentration decreased with increasing irrigation rate ( P = 0.002) and soil depth ( P = 0.003), indicating that nitrate leaching to the deepest parts of the soil was enhanced with increased irrigation rates ( Fig. 3 ). Decreased soil nitrate concentration may also be due to high N uptake by the crop, as suggested by augmented vegetative growth with increasing irrigation rate. Nitrate present at 40–60 depth is usually lost as it is not recovered by plants’ roots. Decreased nitrate in 40- to 60-cm zone is thus solely due to leaching.

Foliar mineral nutrient concentrations and CI.
In 2010, foliar N and K concentrations decreased and P increased with increasing irrigation rate ( Table 3 ). Other foliar nutrients concentrations were unaffected by irrigation rate. Nitrogen, K, and CI decreased with irrigation rate, possibly as a result of a dilution effect associated with increased aboveground plant growth. In addition, at high irrigation rates plants likely had reduced access to soil N due to increased nitrate leaching. Plant water stress in eggplant can reduce foliar N, P, and K concentrations compared with well-irrigated plants ( Kirnak et al., 2002 ). In the present study, however, only foliar P was reduced at low irrigation rate.
Foliar mineral nutrient concentrations in eggplant as affected by several irrigation rates. Fall of 2010, Tifton, GA. z

Chlorophyll indices have been used as indirect estimators of chlorophyll and leaf N concentrations ( Liu et al., 2006 ). Crop drought stress may influence leaf morphology (e.g., increased specific leaf weight) in plants ( Larcher, 1995 ); these variations in leaf morphology may also influence CI, making difficult to use CI to estimate leaf N ( Díaz-Pérez, 2013 ). In our study, CI values increased with increasing leaf N ( R 2 = 0.921; P = 0.001), supporting the use of chlorophyll meter to estimate leaf N.
In 2010, fruit number and fruit yields (marketable and total) were lowest at 33% ETc and there were little yield differences among irrigation rates higher than 33% ETc ( Fig. 4 ). Individual fruit weight was also reduced at 33% ETc ( Fig. 5 ). There was a higher correlation between fruit number and fruit yield ( R 2 = 0.94; P < 0.0001) than between individual fruit weight and fruit yield ( R 2 = 0.15; P = 0.027), suggesting that marketable yield was determined more by fruit number than individual fruit weight. In greenhouse-grown eggplant, soil water deficit decreased fruit number but not fruit size ( Chartzoulakis and Drosos, 1995 ). In a study with different levels of irrigation and N fertilizer, eggplant fruit yield was more related with fruit number than with fruit size ( Aujla et al., 2007 ). In another study, soil water deficits also reduced eggplant fruit size, but the effect of drought stress on fruit number was not evaluated ( Kirnak et al., 2002 ). In 2011, irrigation rate had no effect on the number or yields of marketable, cull, and total fruit, or on individual fruit weight ( Table 4 ). There were no significant interactions between harvest dates and irrigation rates. There was also a higher correlation between fruit number and fruit yield ( R 2 = 0.92; P < 0.0001) than between individual fruit weight and fruit yield ( R 2 = 0.185; P = 0.001). Results suggest that eggplant may tolerate moderate water stress, since plants irrigated at 67% ETc produced fruit yields similar to those of plants irrigated at 100% ETc or higher rates. Thus, there is a potential to reduce irrigation rates below 100% ETc without negatively impacting fruit yields.

Fruit yield of eggplant as affected by irrigation rate. Fall of 2011, Tifton, GA.

Irrigation water use efficiency and agronomic efficiency of nitrogen.
Plants received more irrigation water in 2010 than in 2011 as a result of reduced rainfall in 2010 ( Table 5 ). In both years, IWUE decreased with increasing irrigation rate. IWUE was greatly reduced and there were significant effects of irrigation rates on several variables in 2010, but not in 2011. Increased IWUE and increased SWC in 2011 (mean = 13.4% at 0- to 12-cm depth) relative to SWC in 2010 (mean = 7.5% at 0- to 12-cm depth) are probably associated with increased contribution of soil water from rainfall and drainage water from upper areas of the field; in 2011, field used was low and nearly flat.
Irrigation, cumulative rainfall, IWUE, and AEN of eggplant crop grown on plastic film mulch. Fall of 2010 and 2011, Tifton, GA.

Although there were differences in leaf N among irrigation treatments, fruit yield was likely more related to irrigation rate than to leaf N. Total yield showed a quadratic relationship with leaf N ( R 2 = 0.185; P = 0.013); total yield was unaffected by leaf N below 5.1% and was lowest at the highest leaf N (5.3%) occurred at the lowest irrigation rate (33% ETc).
Agronomic efficiency of N increased with irrigation rate in 2010 likely as a result of increased fruit yield associated with improved plant water status; AEN was unaffected by irrigation rate in 2011. AEN values in this study (range 92 to 187 kg·kg −1 N) were lower compared with values of other studies on eggplant (range = 324 to 859 kg·kg −1 N) ( Aujla et al., 2007 ), probably because the harvest period in this study was reduced. Low AEN values may also mean that eggplant crop in this study made inefficient use of N fertilizer, probably in part due to overfertilization. Aujla et al. (2007) reported that irrigation rate and N fertilization rate interacted in drip-irrigated eggplants; they also found that irrigation at 75% pan evaporation and 120 kg·ha −1 N produced the greatest yields, and that AEN increased with increased N fertilization rate.
Fruit DW content and HI.
In year 2010, fruit DW content (mean = 6.2%) was unaffected by irrigation rate. In a study under semiarid conditions, soluble DW or soluble solids in eggplant decreased with increased irrigation rates ( Kirnak et al., 2002 ). In greenhouse-grown eggplant, increased irrigation rates also decreased fruit DW content ( Chartzoulakis and Drosos, 1995 ).
Harvest index was unaffected by irrigation rate (mean HI = 0.32). These data suggest that eggplant is more tolerant to drought than other solanaceous crops ( Behboudian, 1977 ). Our measurements of HI did not include root biomass. However, under water stress, eggplants possibly allocated increased amounts of assimilates for root growth as occurs in other plants ( Larcher, 1995 ). In habanero pepper, an irrigation rate of 20% of available water produced reduced values of HI ( Quintal Ortiz et al., 2012 ). In tomato, there was no difference in total dry biomass and HI between the control and a partial irrigation treatment, but total dry biomass and HI significantly decreased under regulated deficit irrigation ( Lei et al., 2009 ); moderate water stress–induced osmotic regulation under partial root drying conditions, leading to normal water status and the same level of biomass. Eggplant in our study was also able to maintain high fruit yields at moderate levels of water stress, suggesting that, as tomato, eggplant is also able to develop mechanisms to deal with water stress such as osmoregulation.
In conclusion, the results from this research indicate that eggplant may tolerate moderate water stress, since plants irrigated at 67% ETc had no detrimental effects on plant growth and leaf gas exchange and produced fruit yields similar to those of plants irrigated at 100% ETc. Thus, there is a potential to reduce current irrigation rates without negatively impacting fruit yields or quality.
Literature Cited
Allen, R.G. , Pereira, L.S. , Raes, D. & Smith, M. 1998 Crop evapotranspiration: Guidelines for computing crop water requirements. Food and Agriculture Organization of the United Nations, Rome
Aujla, M.S. , Thind, H.S. & Buttar, G.S. 2007 Fruit yield and water use efficiency of eggplant ( Solanum melongema L.) as influenced by different quantities of nitrogen and water applied through drip and furrow irrigation Sci. Hort. 112 142 148
- Search Google Scholar
- Export Citation
Behboudian, M.H. 1977 Responses of eggplant to drought. I. Plant water balance Sci. Hort. 7 303 310
CAED 2013 2012 Georgia farm gate value report. CAES, Univ. of Georgia, Athens, GA
Calhoun, J.W. 1983 Soil survey of Tift County, Georgia. United States Department of Agriculture, Soil Conservation Service, University of Georgia, GA
Chartzoulakis, K. & Drosos, N. 1995 Water use and yield of greenhouse grown eggplant under drip irrigation Agr. Water Mgt. 28 113 120
Díaz-Pérez, J.C. 2013 Bell pepper ( Capsicum annum L.) crop as affected by shade level: Microenvironment, plant growth, leaf gas exchange, and leaf mineral nutrient concentration HortScience 48 175 182
Díaz-Pérez, J.C. , Granberry, D. , Seebold, K. , Giddings, D. & Bertrand, D. 2004 Irrigation levels affect plant growth and fruit yield of drip-irrigated bell pepper HortScience 39 748
Gaveh, E.A. , Timpo, G.M. , Agodzo, S.K. & Shin, D.H. 2011 Effect of Irrigation, transplant age and season on growth, yield and irrigation water use efficiency of the African eggplant J. Hort. Environ. Biotechnol. 52 13 28
Jaimez, R.E. , Rada, F. & Garcia-Nunez, C. 1999 The effect of irrigation frequency on water and carbon relations in three cultivars of sweet pepper ( Capsicum chinense Jacq), in a tropical semiarid region Sci. Hort. 81 301 308
Karam, F. , Saliba, R. , Skaf, S. , Breidy, J. , Rouphael, Y. & Balendonck, J. 2011 Yield and water use of eggplants ( Solanum melongena L.) under full and deficit irrigation regimes Agr. Water Mgt. 98 1307 1316
Kirnak, H. , Tas, I. , Kaya, C. & Higgs, D. 2002 Effects of deficit irrigation on growth, yield, and fruit quality of eggplant under semi-arid conditions Austral. J. Agr. Res. 53 1367 1373
Larcher, W. 1995 Physiological plant ecology: Ecophysiological and stress physiology of functional groups. Springer, Berlin, Germany
Lei, S. , Yunzhou, Q. , Fengchao, J. , Changhai, S. , Chao, Y. , Yuxin, L. , Mengyu, L. & Baodi, D. 2009 Physiological mechanism contributing to efficient use of water in field tomato under different irrigation Plant Soil Environ. 55 128 133
Liu, Y.J. , Tong, Y.P. , Zhu, Y.G. , Ding, H. & Smith, E.A. 2006 Leaf chlorophyll readings as an indicator for spinach yield and nutritional quality with different nitrogen fertilizer applications J. Plant Nutr. 29 1207 1217
Locascio, S.J. , Olson, S.M. & Rhoads, F.M. 1989 Water quantity and time of N and K application for trickle-irrigated tomatoes J. Amer. Soc. Hort. Sci. 114 265 268
Madramootoo, C.A. & Rigby, M. 1991 Effects of trickle irrigation on the growth and sunscald of bell peppers ( Capsicum annuum L.) in southern Quebec Agr. Water Mgt. 19 181 189
Ngouajio, M. , Wang, G.Y. & Goldy, R. 2007 Withholding of drip irrigation between transplanting and flowering increases the yield of field-grown tomato under plastic mulch Agr. Water Mgt. 87 285 291
Ozores-Hampton, M. 2014 Conventional and specialty eggplant varieties in Florida. Horticultural Sciences Department, Univ. of Florida/Institute of Food and Agricultural Sciences. Document HS1243. 12 June 2015. < http://edis.ifas.ufl.edu/hs1243 >.
Pereira, L.S. , Allen, R.G. , Smith, M. & Raes, D. 2015 Crop evapotranspiration estimation with FAO56: Past and future Agr. Water Mgt. 147 4 20
Quintal Ortiz, W.C. , Perez-Gutierrez, A. , Latournerie Moreno, L. , May-Lara, C. , Ruiz Sanchez, E. & Martinez Chacon, A.J. 2012 Water use, water potential, and yield of habanero pepper ( Capsicum chinense Jacq.) Rev. Fitotec. Mex. 35 155 160
Sarker, B.C. , Hara, M. & Uemura, M. 2005 Proline synthesis, physiological responses and biomass yield of eggplants during and after repetitive soil moisture stress Sci. Hort. 103 387 402
Sezen, S.M. , Yazar, A. & Eker, S. 2006 Effect of drip irrigation regimes on yield and quality of field grown bell pepper Agr. Water Mgt. 81 115 131
Simonne, E.H. , Dukes, M.D. , Hochmuth, R.C. , Studstill, D.W. , Avezou, G. & Jarry, D. 2006 Scheduling drip irrigation for bell pepper grown with plasticulture J. Plant Nutr. 29 1729 1739
USDA 2013 United States standards for grades of eggplant. United States Department of Agriculture
Contributor Notes
Financial support was provided by the Georgia Agricultural Experiment Stations.
We thank John Silvoy, Jesús Bautista, and Nélida Bautista for their invaluable technical support. We also thank Peter Germishuizen from Lewis Taylor Farms, Ty Ty, GA, for donation of eggplant transplants. We appreciate the thorough review of the manuscript by Pat Conner, Tim Coolong, Erick Smith, and the anonymous reviewers.
Mention of trade names in this publication does not imply endorsement by the University of Georgia of products named, nor criticism of similar ones not mentioned. The cost of publishing this paper was defrayed in part by payment of page charges. Under postal regulations, this paper therefore must hereby be marked advertisement solely to indicate this fact.
1 Corresponding author. E-mail: [email protected] .
Headquarters:
1018 Duke Street
Alexandria, VA 22314
Phone : 703.836.4606
Email : [email protected]
© 2018-2024 American Society for Horticultural Science
- [66.249.64.20|81.177.182.154]
- 81.177.182.154
Character limit 500 /500
ORIGINAL RESEARCH article
Evaluation of stripe rust resistance and genome-wide association study in wheat varieties derived from the international center for agricultural research in the dry areas.
- 1 School of Ecological and Environmental Engineering, Qinghai University, Xining, Qinghai, China
- 2 Qinghai Academy of Agriculture and Forestry Science, Qinghai University, Xining, Qinghai, China
- 3 National Crop Germplasm Resources Duplicate, Xining, Qinghai, China
159 wheat varieties obtained from ICARDA, CYR32, CYR33 and CYR34 were used to evaluate the stripe rust resistance in this study. Seedling resistance was carried out in the green house at the two-leaf stage. Adult-plant resistance was carried out between 2022 and 2023 in Xining and Guide, respectively. A total of 24,151 high-quality SNP loci were obtained from a 55K SNP chip data. Genome-wide association study was carried out between SNP loci and stripe rust resistance. Seedling resistance screening revealed that 91.8% (146) of wheat varieties were resistant to CYR32 and CYR33, while only 49.7% (79) of wheat varieties were resistant to CYR34. Adult-plant resistance showed 153 (96.2%) germplasms represented resistance in 2022, while only 85 (53.4%) showed resistance in 2023. An association study using the 55K SNP chip data results combined with disease ratings of 159 materials at both the seedling and adult stages discovered 593 loci related to stripe rust resistance (P ≤ 0.0001). These loci exhibited contribution rates ranging from 11.1% to 18.7%. Among them, 71 were significantly related to resistance against CYR32 at the seedling stage, with a contribution rate of 12.7%-17.2%. Constituting the vast majority, 518 loci distributed across 21 chromosomes were significantly related to CYR33 at the seedling stage, with a contribution rate of 12.6%-18.7%. Fewer loci were found to be associated with disease resistance in adult plants. In E1 environment, a sole locus was detected on chromosome 2B with a contribution rate of 14.4%. In E2 environment, however, exhibited three loci across chromosomes 2B, 4A, and 7B with contribution rates ranging from 11.1% to 16.9%. A total of 68 multi-effect loci were significantly related to resistance against both CYR32 and CYR33 at the seedling stage, and one stable locus was significantly associated with stripe rust resistance at the adult plant stage.
1 Introduction
As one of the world’s most important cereal crops, wheat plays a central role in global food security ( Juliana et al., 2017 ). Wheat stripe rust is prevalent in China but is especially troublesome in some provinces such as Qinghai, Guizhou, Shaanxi, Shanxi, Chongqing, and Sichuan ( Dong et al., 2019 ). The disease is recognized as a significant threat to Chinese food security due to its wide distribution range, rapid transmission, and capacity to cause substantial yield losses ( Wang et al., 2016 ; Dai et al., 2019 ). Since its initial discovery in Europe in 1777, the pathogen has been reported in over 60 countries ( Line, 2002 ; Chen, 2005 ).Recently, scientists have reported a total of 86 ( Yr1 - Yr86 ) stripe rust resistance genes distributed across all chromosomes except chromosome 1A ( Klymiuk et al., 2022 ). An additional 100 genes have been tentatively named but await official adoption. Moreover, researchers have mapped over 300 QTLs controlling wheat stripe rust resistance ( Li et al., 2020 ; Feng et al., 2023 ; Zhu et al., 2023 ). Previous virulence analyses revealed that only Yr5 and Yr15 confer high resistance against races CYR32, CYR33, and CYR34 throughout the whole wheat growth period, while Yr10 , Yr24 , and Yr26 are only effective against CYR32 and CYR33. Yr9 , Yr18 , and Yr41 were susceptible against all three races during both the seedling and adult stages ( Zeng et al., 2015 ; Wang et al., 2019 ). Previous research has tested thousands of wheat production varieties, reserve varieties, high-generation strains, and disease-resistant source materials for reactions to stripe rust at the adult plant stage. Many varieties previously considered resistant to wheat stripe rust were found to be susceptible to CYR34, indicating that the pathotype exhibits strong pathogenicity and high parasitic potential. These findings suggested that CYR34 posed a serious threat to wheat production in China ( Huang et al., 2018 ). Furthermore, a new physiological race of stripe rust, tentatively named “TAS-6”, has been reported to overcome resistance conferred by Yr5 ( Zhang et al., 2020 ). Therefore, uncovering new sources of genetic disease resistance for use in wheat breeding is the key to ensuring global food security ( Xu et al., 2020 ).
Plant breeders often rely on Genome-Wide Association Studies (GWAS), which allow them to analyze the genetic basis of traits at the population level by testing the significance of the association between genetic markers and phenotypic variations ( Liu et al., 2017 ). GWAS has quickly become popular with plant researchers due to its faster results, greater breadth, and higher accuracy since 2001 ( Thornsberry et al., 2001 ; Flint-Garcia et al., 2005 ). In 2006, researchers effectively used a GWAS to discover influential QLTs in wheat for the first time ( Breseghello and Sorrells, 2006 ). The widespread use of the technology has broadened our understanding of the plant. To date, a total of 37 genes/QTLs, including 10 potential new QTLs, have been detected using 616 spring wheat varieties and breeding lines ( Liu L. et al., 2020 ). New disease resistance loci have been discovered through GWAS by combining 9K SNPs with phenotypic data at the adult-plant stage ( Maccaferri et al., 2015 ). In a previous association study utilizing 660K SNPs from 411 spring wheat lines grown over multiple years and environments, researchers identified TraesCS2B01G513100, a candidate gene that is associated with stripe rust resistance. The researchers then developed molecular markers to assist with disease resistance breeding ( Wu et al., 2020 ). Based on 90K SNP chip data, a separate association study of 375 natural wheat varieties from home and abroad identified 26, 22, and 25 significantly associated SNP loci on the short arms of 2AS, 2BS, and 2DS in the second homology group, respectively. These loci can explain 4.61%-11.75% of the observed phenotypic variation ( Zhang et al., 2019 ). Taken together, the results of these studies provide a basis for the cultivation of new stripe rust-resistant wheat varieties and offer guidance for the discovery of new genes.
ICARDA’s (the International Center for Agricultural Research in the Dry Areas) mission is to address challenges caused by harsh and ever-changing environments; and ICARDA’s breeding program also emphasizes the importance of utilizing varieties with local adaptability, as well as the necessity of plant biodiversity for human survival; in addition, ICARDA preserves thousands of wheat crop resources, which provides necessary conditions for us to introduce wheat materials from ICARDA and cultivate new varieties ( Lu, 2022 ). With the continuous development of molecular markers and the emergence of new technologies, novel stripe rust resistance genes are becoming more plentiful and more accessible for wheat breeders ( Luo et al., 2010 ). Various molecular marker techniques have been adopted by researchers to analyze stripe rust resistance and to discover new resistance loci ( Bulli et al., 2016 ; Liu et al., 2018 ). Recently, there is little molecular-based research regarding agronomic traits, disease resistance, and stress resistance in introduced wheat varieties in Qinghai Province. In this study, we conducted a GWAS using introduced ICARDA wheat varieties and 55K SNP to uncover potentially significant disease resistance loci in both wheat seedlings and adult plants, which offered a theoretical reference for the stripe rust resistance of ICARDA wheat varieties and excavating new stripe rust resistance genes or QTLs.
2 Materials and methods
2.1 test materials.
159 wheat varieties derived from ICARDA were obtained and designated as ICARDA001-ICARDA159 ( Supplementary Table 1 ). CYR32, CYR33, and CYR34 were provided by the wheat stripe rust breeding laboratory of Northwest A&F University.
2.2 Disease resistance evaluation at the seedling stage
To assess disease resistance in wheat seedling stage, 10 seeds of a wheat variety were sown in a container and grown under suitable conditions. Once the plants reached the two-leaf stage, they were uniformly sprayed with a 1:100 ratio of Tween to water. Next, each physiological race of stripe rust was mixed with talc in a 1:20 ratio and shaken onto the test varieties. After the wheat leaves were completely diseased, the infection type was rated according to Line and Qayoum’s 9-level reaction pattern.
2.3 Disease resistance evaluation at the adult-plant stage
The stripe rust resistance of adult wheat was evaluated between 2022 and 2023 in experimental fields located in Xining and Guide of Qinghai Province. In 2022, trials in Xining and Guide were designated E1 and E2, respectively, and were inoculated with a mixture of CYR32 and CYR33. In 2023, trials in Xining and Guide were designated as E3 and E4, respectively, and were inoculated with a mixture of CYR32 and CYR34. Wheat lines were scored by recording the number of rust lesions on each leaf during the height of infection.
2.4 Genotypic analysis
The genome of 159 wheat varieties was analyzed using a 55K SNP chip developed by the Affymetrix Axiom platform, provided by ZhongYuJin Marker (Beijing) Biotechnology Co., Ltd. Affymetrix Axiom Analysis Suite software (Thermo Fisher) was employed for preliminary screening and genotyping. A total of 53,063 loci were obtained from the raw data, following criteria of DQC (Data Quality Control) of ≥0.82 and CR (Call Rate) of ≥0.95 ( https://datadryad.org/stash/share/aphTKrewmqNQnQgdj7vgUlw7I8jPMCT0GYiCyDNneSw ). VCFtools software ( Danecek et al., 2011 ) was used for site filtering with a missing rate of >20% and an MAF (Minor Allele Frequency) <0.05, resulting in 24,151 high-quality SNP sites. The marker density map for the obtained high-quality SNP loci was plotted using the CMplot package in the R suite.
2.5 Genetic diversity and population structure
The genetic diversity was calculated with PowerMarker V3.25 software ( Liu and Muse, 2005 ) using the obtained 24,151 high-quality SNP sites. These calculations adhered to the PIC (Polymorphic Information Content) parameter, represented by PIC=1-Σ(P ij ) 2 (P ij represents the frequency of the j th allele at the i locus). Plink 1.9 software ( Purcell et al., 2007 ) was used to perform LD filtering on SNP sites, resulting in 2,821 markers. Admixture software ( Alexander et al., 2009 ) was used to analyze the population structure. The range of K values for the subgroups was preset to be 2-15, and the average value was calculated based on six repetitions. The average Cross-Validation ( CV ) value was calculated for each K value, and the K value associated with the lowest CV value was selected as the optimal subgroup number. Finally, a subgroup map was drawn using R software.
2.6 GWAS of stripe rust resistance
A GWAS was conducted on the 159 wheat varieties using 24,151 high-quality SNPs, phenotypic stripe rust resistance data collected at both the seedling and adult plant stages, a kinship matrix (K-matrix) generated by Tassel 5.0 software ( Bradbury et al., 2007 ) and a Q-matrix created by Admixture.
3 Results and analysis
3.1 stripe rust resistance identification at seedling and adult-plant stages.
During disease resistance screening of wheat seedlings, 21 (13.2%) varieties showed immunity to CYR32, while 125 (78.6%) varieties showed high resistance to CYR32, the remaining 13 (8.2%) lines showed susceptibility to rust stripe race CYR32, including IR002, IR017, IR037, IR053, IR055, IR061, IR073, R077, IR078, IR125, IR128, IR135, and IR149. For CYR33, 18 (11.3%) varieties showed immunity, 128 (80.5%) wheat materials showed high resistance, 1 (0.6%, IR133) varieties showed moderate resistance, and 12 (7.6%) varieties showed susceptibility (except for IR135 among the 13 materials mentioned above). For CYR34, 11 (6.9%) materials showed immunity, 68 (42.8%) materials showed high resistance, 1 (0.6%, IR107) material showed moderate resistance, and 79 (49.7%, including the 13 materials aforementioned) materials showed susceptibility, and most of them are highly susceptible to diseases ( Supplementary Table 2 ; Figure 1 ). With the pervalent race of CYR34, most wheat varieties lost resistance at the seedling stage.
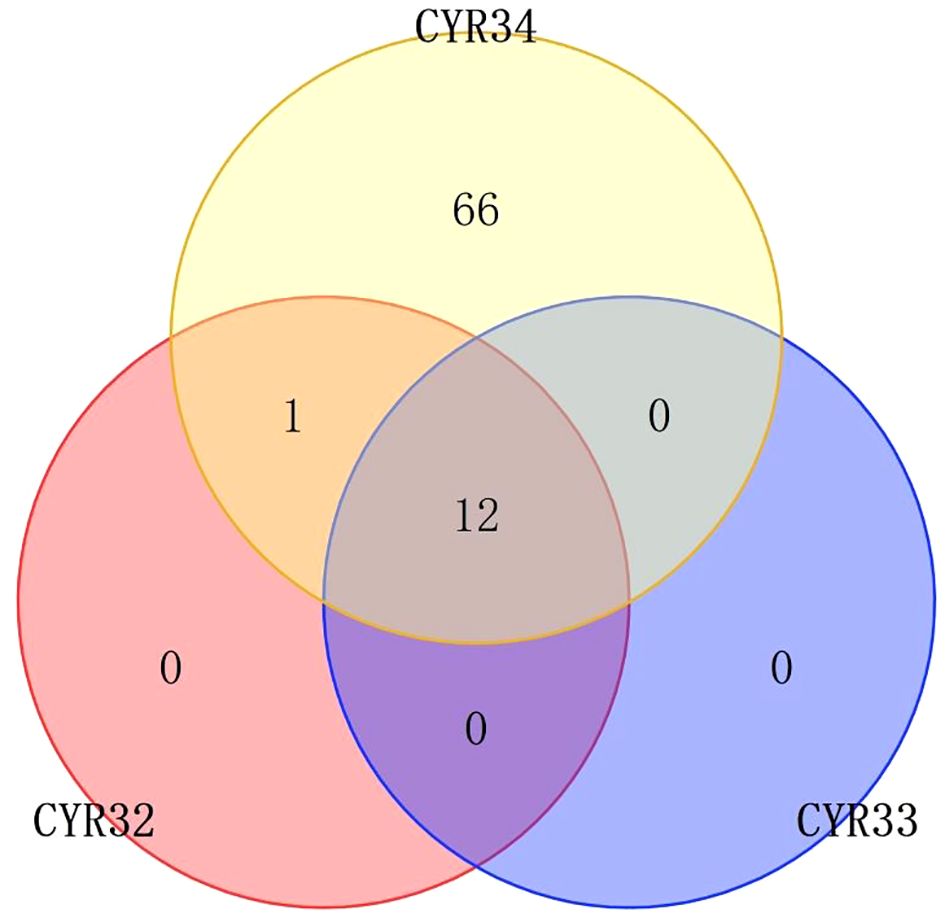
Figure 1 Venn plot of resistance distribution of wheat materials to CYRThe numbers in the figure represent the number of wheat materials that are resistant to this CYR, and the overlap represents the number of wheat materials that are resistant to both two or three CYR species.
For the stripe rust evaluation of the adult plant stage in Xining, under the E1 environment, 6 materials (3.8%, IR017, IR055, IR125, IR128, IR135, and IR149) showed susceptibility, 111 materials (69.8%) showed high resistance, and 42 materials (26.4%) showed moderate resistance; in the E3 environment, 74 (46.5%) materials showed susceptibility, of which 68 showed resistance in 2022, 72 (45.3%) showed high resistance, and 13 (8.2%) showed moderate resistance. Compared with the E1and E3 environment, there were 50 materials (31.4%) with enhanced disease resistance in the E3 environment, 12 materials (7.5%) with unchanged resistance, and 23 materials (14.5%) with reduced resistance ( Supplementary Table 3 ).
For the identification results of the adult plant stage in Guide, under E2 environment, 6 (3.8%, the same as E1 environment) materials showed susceptibility, 113 (71.1%) materials showed high resistance, and 40 (25.2%) materials showed moderate resistance; in the E4 environment, 74 (45.3%, similar to the E3 environment) materials showed susceptibility, of which 68 materials showed resistance in 2022, 66 (41.5%) materials showed high resistance, and 19 (11.9%) materials showed moderate resistance. Compared with E2 and E4 environment, there were 49 materials (30.8%) with enhanced resistance in the E4 environment, 12 materials (7.5%) with unchanged resistance, and 24 materials (15.1%) with reduced resistance ( Supplementary Table 3 ).
3.2 Genotype analysis
The SNP density map illustrated a non-uniform distribution of 24,151 high-quality SNP loci across three genomes and 21 chromosomes, there was a portion of fragments without SNP markers, and the density was almost entirely concentrated at 5-15 loci/Mb. Among them, the SNP density on chromosome 3D was the highest at 1.7424/Mb, followed by 4D chromosome at 1.4263/Mb, and chromosome 6A has the lowest density at 0.3982/Mb; the number of SNPs on chromosome 4B was the highest, with 1675, followed by chromosome 5B with 1652, and the smallest was chromosome 4D with only 357. There were 9,338 (38.7%), 9,865 (40.8%), and 4,948 (20.5%) polymorphisms identified in genomes A, B, and D respectively. The overall polymorphism ratio was 45.5% (2,4151/53,063), with the highest occurrence in genome B, followed by A and then D. Among them, chromosome 2A possessed the highest PIC value of 0.3526, followed by chromosome 4A with a PIC value of 0.3467. The lowest PIC value was chromosome 3B with 0.2433; the PIC value of the genome A was the highest at 0.3264, followed by the B at 0.2999, and D chromosome at 0.2937. The average PIC value of all chromosomes is 0.3067 ( Table 1 ; Figure 2 ).
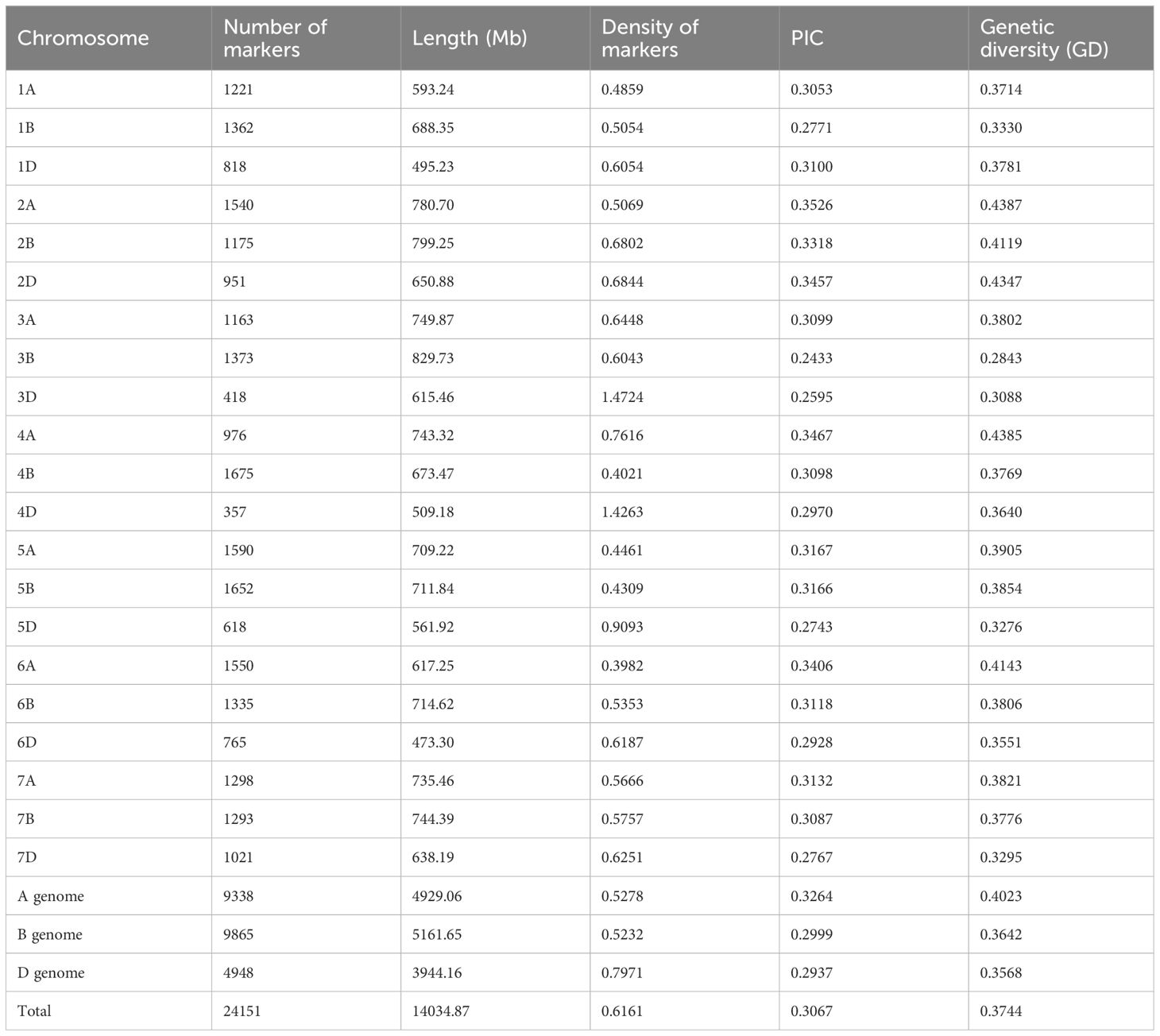
Table 1 SNP markers and polymorphisms of 159 wheat materials.
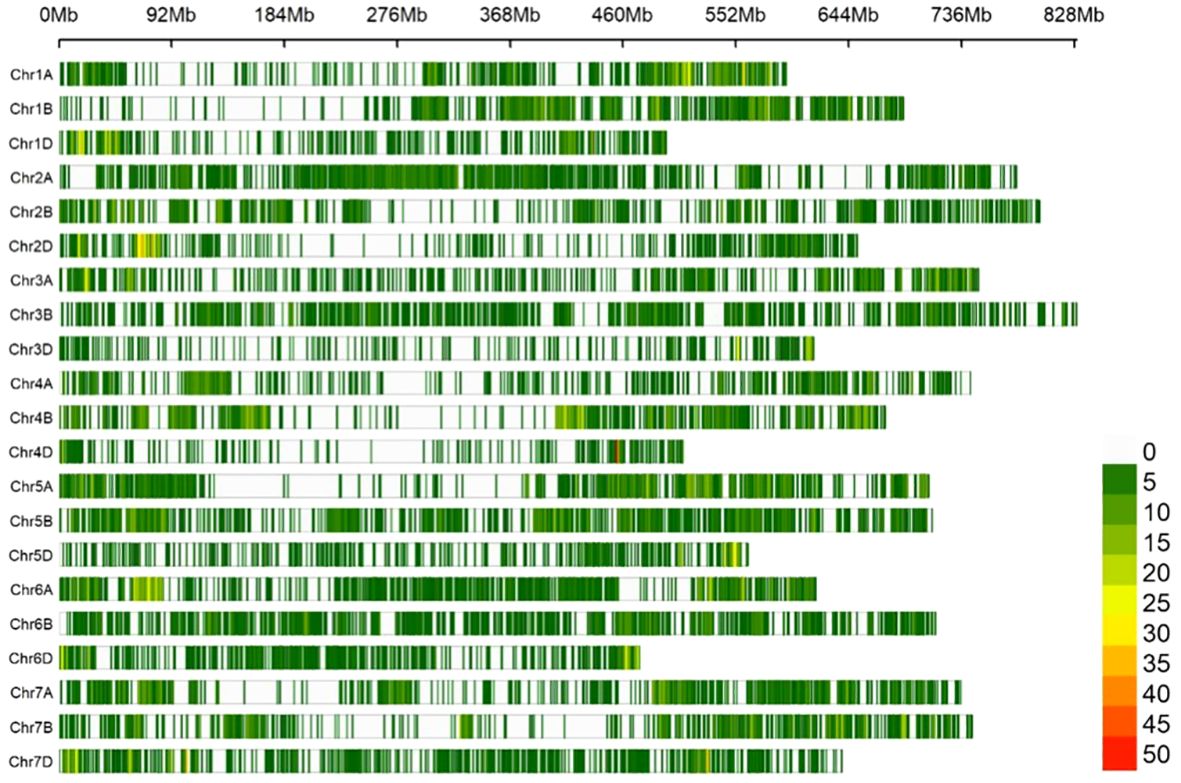
Figure 2 SNP density map. Indicates the number of SNPs per 1Mb of length on each chromosome, and different colors indicate different SNP densities.
3.3 Genetic diversity and population structure
The analysis of genetic diversity (GD) revealed an average GD of 0.3744 among the 159 wheat lines, with variations ranging from 0.284 3to 0.4387. Genome A demonstrated the highest GD, followed by B and D. Among all chromosomes, the 2A chromosome had the highest GD value of 0.4387, followed by the 4A chromosome with a GD value of 0.4385, and the 3B chromosome had the lowest value of only 0.2843.
The population structure analysis of the 159 wheat materials revealed that at the minimum CV value, K=11, suggesting that the materials could be divided into 11 subgroups. The Q-matrix generated when K=11 was employed as the covariate in the subsequent association study. The subgroups were comprised of the following: subgroup I with 7 (4.4%) varieties; subgroup II with 21 (13.2%) varieties; subgroup III with 16 (10.1%) varieties; subgroup IV with 11 (6.9%) varieties; subgroup V with 12 (7.6%) varieties; subgroup VI with 18 (11.3%) varieties; subgroup VII with 15 (9.4%) varieties; subgroup VIII with 22 (13.8%) varieties, subgroup IX with 4 (2.5%) varieties; subgroup X with 10 (6.3%) varieties; and subgroup XI with 23 (14.5%) varieties ( Figure 3 ).
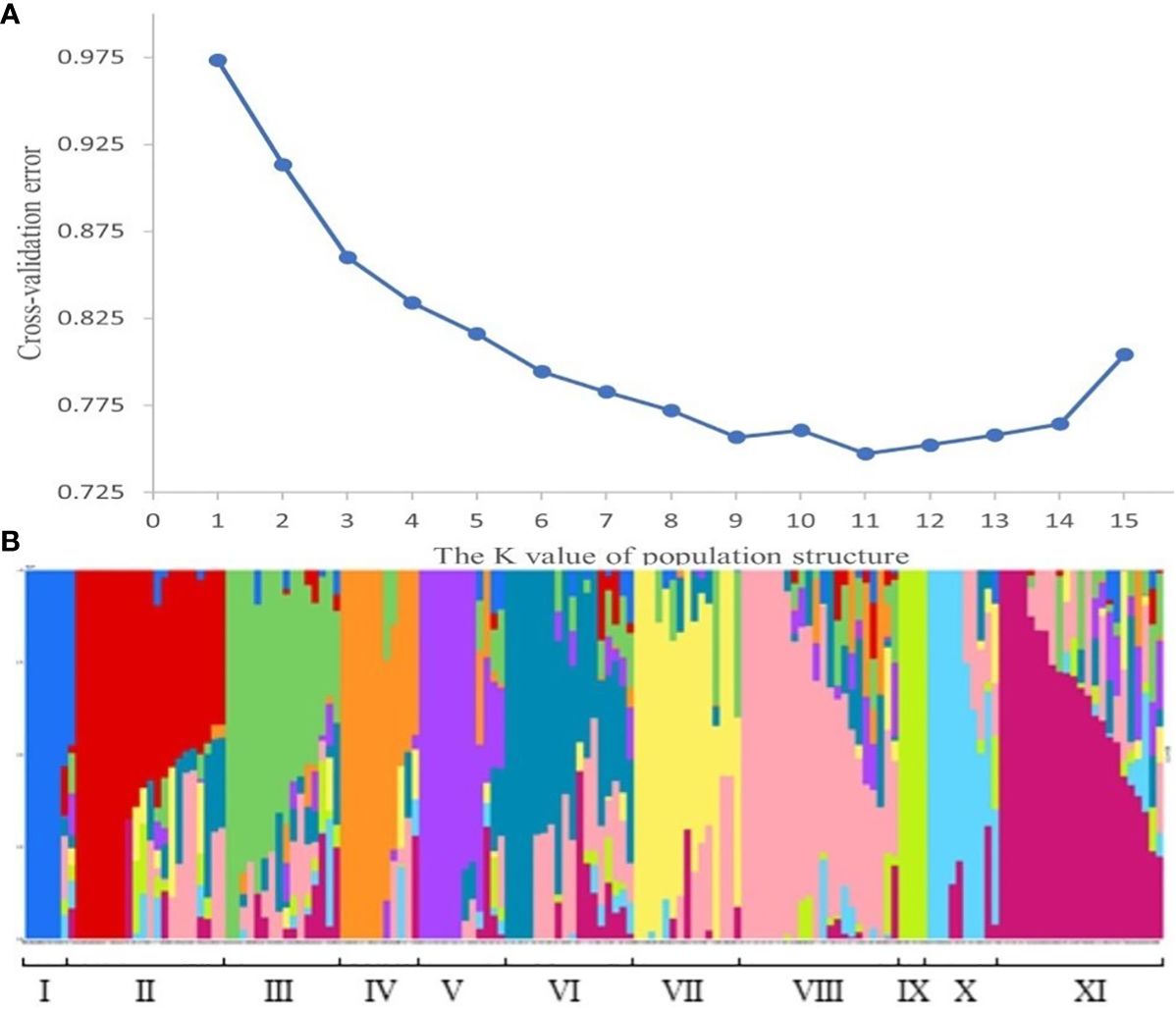
Figure 3 Population structure. (A) represents the CV value for the number of subgroups. The abscissa is the K value of the population structure, and the ordinate is the CV value corresponding to the K value, and the figure shows that when K=11, the CV value is the smallest, that is, 11 is the optimal number of subgroups. (B) represents the composition of each subgroup. Each histogram represents a wheat material. The color and proportion of this histogram indicates which subgroup the material belongs to and what the proportion of descent it belongs to.
3.4 Association study
The association study of ICARDA wheat varieties using 24,151 high-quality SNP loci and phenotypic data identified 593 loci associated with stripe rust resistance, contributing rates ranging from 11.1% to 18.7%. During the seedling stage, 71 loci were found to be related to stripe rust race CYR32. These loci were distributed across chromosomes 1A, 1D, 2A, 2B, 2D, 3A, 3B, 4A, 5A, 5B, 6B, 6D, 7B, and 7D, with contribution rates ranging from 12.7% to17.2%. With the exception of AX-109949596, AX-108783340, and AX-111778082, these loci were also involved in resistance against CRY33 with a contribution rate of 12.6%-17.2%, suggesting that they were multi-effect loci. An additional 518 loci were related to CYR33 and were distributed across all chromosomes with contribution rates of 12.6%-18.7%. In the association study of adult plants, we identified one stable locus, AX-109318462, on chromosome 2B with contribution rates of 14.4%-17.0% in E1 and E2 ( Figures 4 – 6 ; Supplementary Table 4 ). An additional three loci on chromosomes 2B, 4A, and 7B were detected in E2, with contribution rates ranging from 11.1% to 16.9%. No significant loci were detected in E3 and E4. According to the results of the QQ plot, there were loci that were significantly associated with the stripe rust CYR32 and CYR33, E1 and E2 environments in all loci. Although there was a deviation between the observed P value and the expected P value in the QQ plot, which may be due to errors in statistics during the seedling and adult stage phenotype periods by manpower, it can have a certain impact on the results, but overall it can correspond to the results in the Manhattan plot.
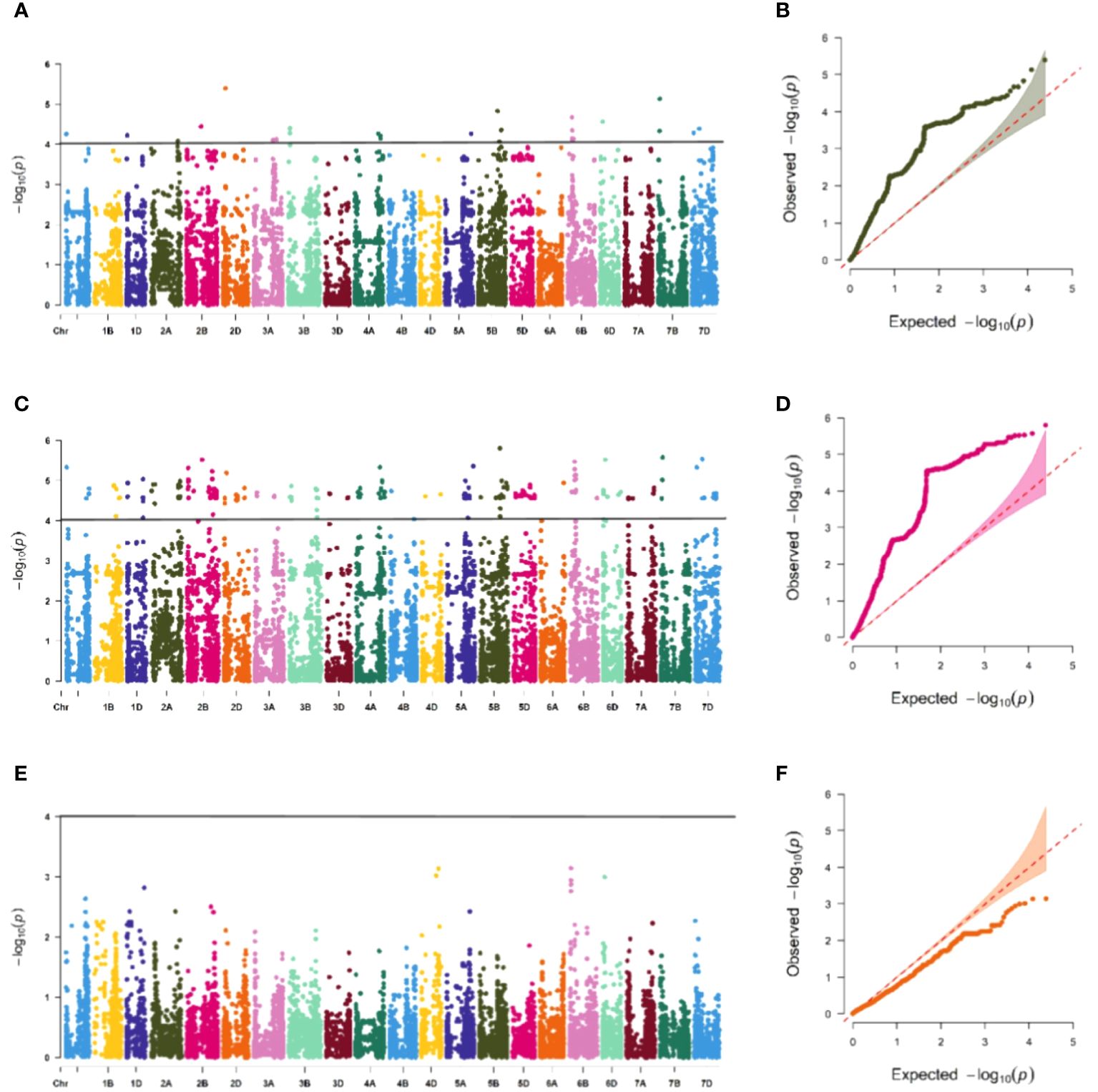
Figure 4 Association study of disease resistance at seedling stage. The Manhattan plot shows the degree of association between SNP loci and traits on each chromosome, and the stronger the association, the higher the height in the figure (left), and the QQ plot shows the consistency between the observed value (ordinate) of P value (ordinate) and the expected value of P value (abscissa), with a significant locus above the dashed line and no significant locus below the dotted line (right). (A, B) are Manhattan plot and QQ plot of CYR32 identification, respectively, and there are loci significantly related to CYR32 on most chromosomes, (C, D) are Manhattan plot and QQ plot of CYR33 identification, respectively, and there are loci significantly related to CYR33 on all chromosomes, and (E, F) are Manhattan plot and QQ plot of CYR34 identification, respectively, and none of the tested materials contain loci that are significantly related to CYR34.
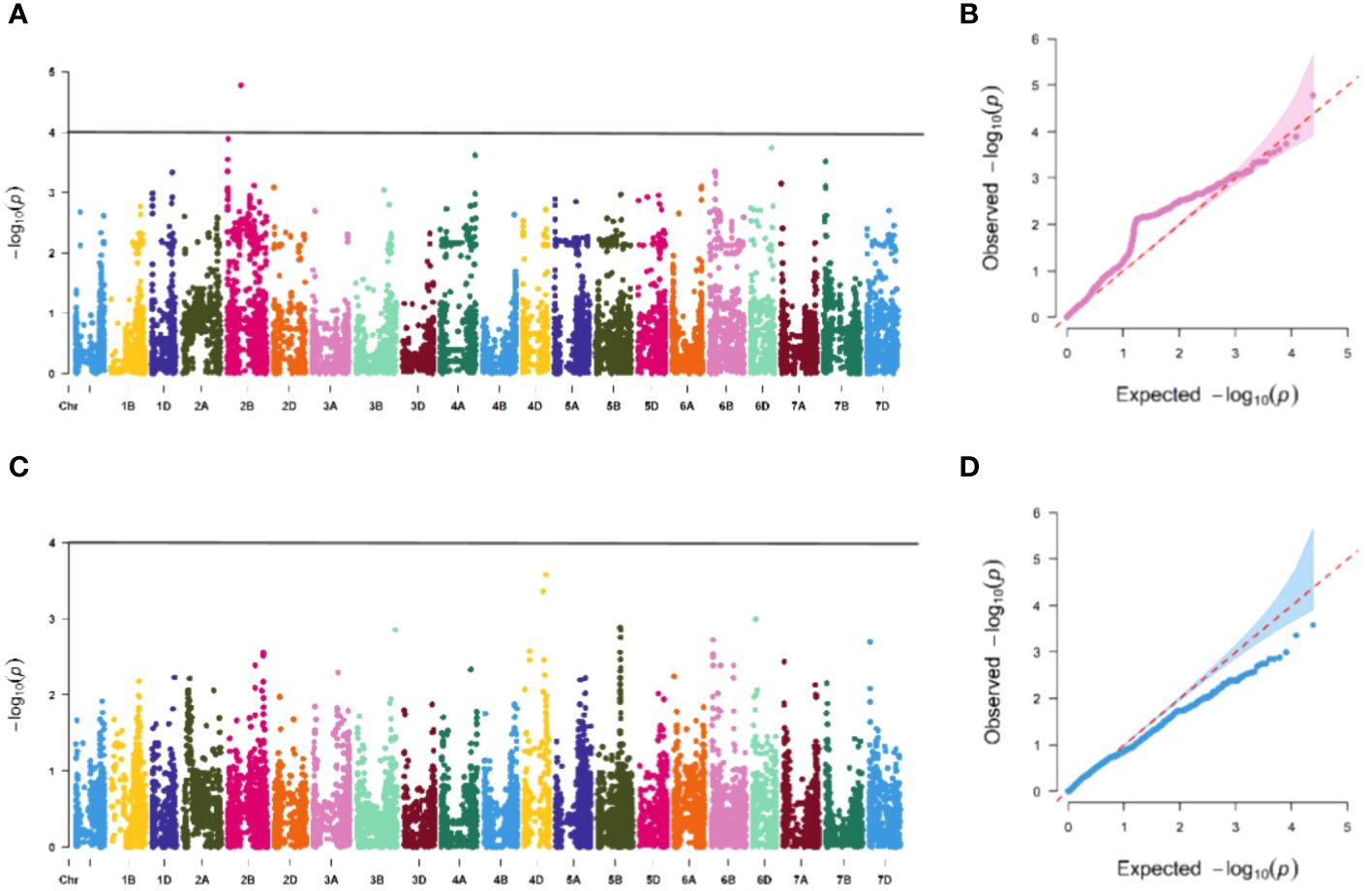
Figure 5 Association study of disease resistance at adult-plant stage in Xining (A, B) are the Manhattan plot and QQ plots of E1, respectively, and only one chromosome has a significant correlation locus, and (C, D) are the Manhattan plot and QQ plot of E3 identification, respectively, and none of the tested materials contain significant correlation.
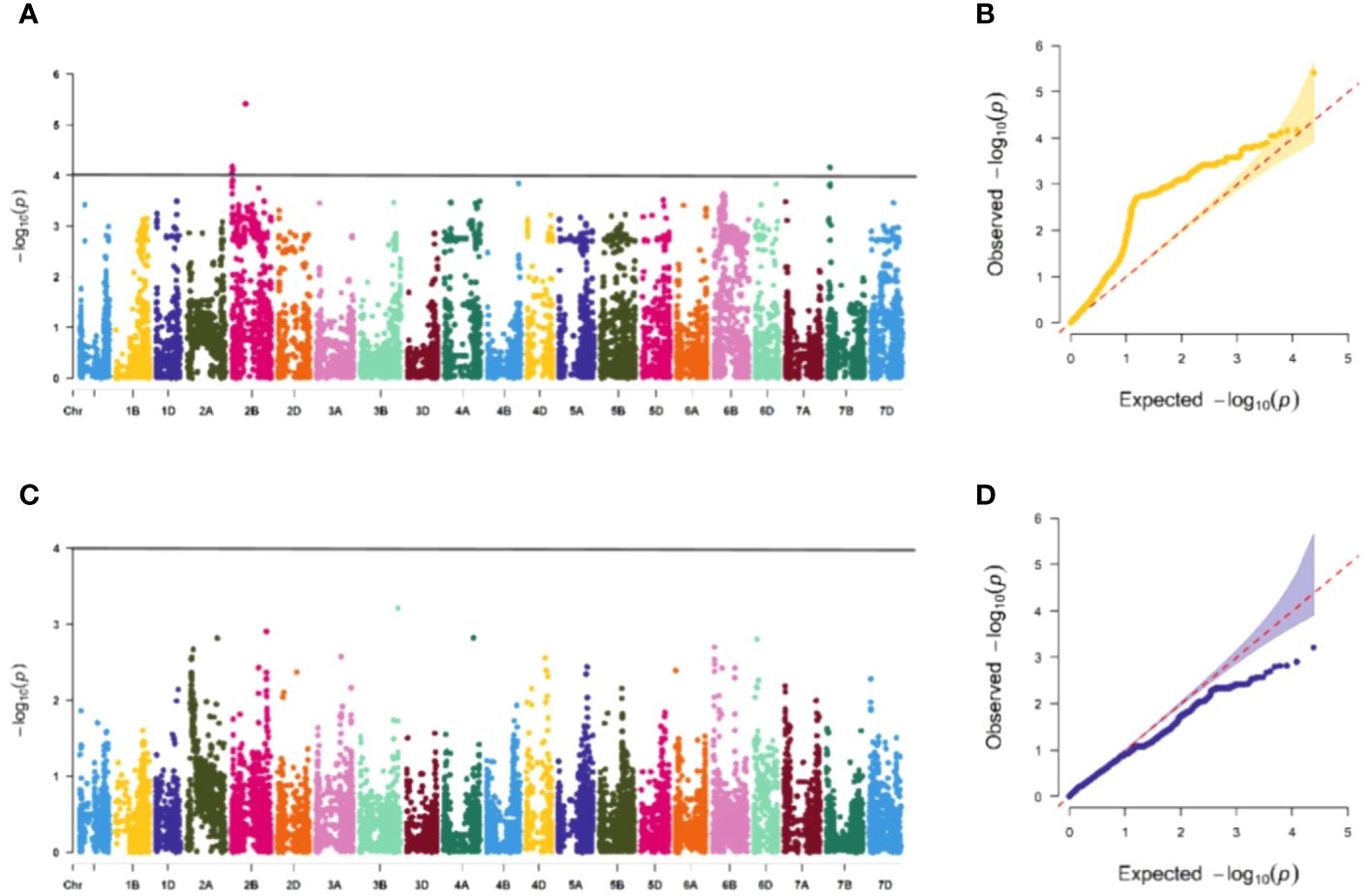
Figure 6 Association study of disease resistance at adult-plant stage in Guide (A, B) are the Manhattan plot and QQ plots of E2, respectively, with a total of 3 significant loci distributed on the 3 chromosomes, and (C, D) are the Manhattan plot and QQ plot of E4 respectively, and none of the test materials contain significant correlation.
4 Discussion
With the global warming and extreme weather, changes in temperature and precipitation have made the harm of stripe rust increasingly aggravated. A recent stripe rust pandemic was reported in 2017, and experts believed that the pandemic was caused by the year’s extremely warm winters, heavy spring rains and a lack of resistance diversity in the main wheat breeding areas ( Zhang, 2022 ). In 2020, wheat stripe rust was included in the list of first-class crop diseases and pests by China, and how to prevent and control effectively is also a key research topic in the world. Therefore, plant protection experts have been constantly updating and improving the management system and technology for many years, but due to the continuous evolution of stripe rust races, abnormal global climate change, etc., the research on stripe rust and disease-resistant varieties will be continuous. At present, the basic and long-term effective means of stripe rust control is the breeding of disease-resistant varieties. However, there is a co-evolution between stripe rust and the host, new types of pathogenic physiological races continue to appear, and the resistance of most wheat varieties in China will be susceptible after 3~5 years of large-scale application, resulting in a disease pandemic ( Chen et al., 2013 ). Due to its special geographical location, Qinghai Province is one of the main summer epidemic areas of wheat stripe rust in China. Because of the influence of climatic conditions, spring wheat is the main wheat plantation, and a large number of stripe rust fungus sources can be found in late spring wheat varieties over summer ( Zhang and Hou, 2022 ). At the same time, since wheat is the main food crop in Qinghai Province, new disease resistance genes were obtained after disease resistance identification and genome-wide association study of wheat materials, which were then introduced into Qinghai Province to enrich the wheat germplasm resources and lay a certain foundation for further wheat breeding research.
4.1 Disease resistance identification
In the screenings of 159 wheat varieties at the seedling stage and during a two-year, two-environment adult field trial, we identified 146 (91.8%) lines that were resistant to both CYR32 and CYR33, while only 80 (50.3%) were resistant to CYR34. These results are consistent with previous studies and suggest that most of the introduced wheat varieties had good resistance to CYR32 and CYR33, indicating that these varieties contained genes, gene combinations and even new genes that could effectively resist CYR32 and CYR33, which could effectively prevent the epidemic of these two epidemic races in the future, and the current materials did not have enough resistance to the new toxic race CYR34 ( Zhang et al., 2022 ). This may be attributed to the early application of CYR32 and CYR33 promoting resistance in wheat ( Zeng et al., 2015 ). Yao conducted a preliminary study on the overwintering conditions of wheat stripe rust in the eastern wheat area of Qinghai, and the results showed that the amount of bacteria before winter was the primary factor affecting the overwintering of wheat stripe rust ( Yao et al., 2014 ). Therefore, the utilization of disease resistance during the whole growth period is of great significance for the prevention and control of stripe rust fungus in Qinghai wheat. Subsequently, these 159 materials can be used for molecular testing to obtain the disease resistance genes that may be contained in them, so as to provide a basis for the development of new varieties.
4.2 Association study of disease resistance
The PIC variation of the 159 materials ranged from 0.243 to 0.353, with an average of 0.307. This indicates that the SNP markers were moderately polymorphic, which is consistent with previous studies ( Kumar et al., 2020 ; Liu YK. et al., 2020 ). Additionally, the genetic diversity ranged from 0.284 to 0.439, with an average of 0.374. This suggests a relatively concentrated genetic background between the breeds, aligning with previous research ( Cao et al., 2015 ; Li et al., 2019 ). An analysis of the 55K SNP chip data from 159 ICARDA wheat varieties combined with the phenotypic stripe rust resistance data revealed that genome B contained the highest quantity of polymorphisms, while genome D exhibited the least. These findings reflect the works of Zhang ( Zhang et al., 2016 ) and Tehseen ( Tehseen et al., 2021 ). A total of 593 significant loci were detected, 68 of which were significant in conferring resistance against both CYR32 and CYR33. These loci are distributed across chromosomes 1A, 1D, 2A, 2B, 2D, 3A, 3B, 4A, 5A, 5B, 6B, 6D, 7B, and 7D. The analysis of stripe rust resistance at the adult-plant stage identified locus AX-109318462 on chromosome 2B as the sole locus associated with resistance in both E1 and E2. Subsequently, referring to the IWGSC_RefSeq_v1.0, the loci within the 5Mb interval on the physical map were considered as one significant locus. Using the QTL genetic map of wheat stripe rust resistance constructed by Cheng ( Cheng et al., 2019 ) and the comparison diagram by Yao (2022) , the 69 loci were compared and analyzed. It was found that 9 of these 69 loci may be newly discovered loci, namely AX-110974432 (3A), AX-110447030 (3A) AX-108736767 (3A), AX-89776892 (3A), AX-110653920 (5B), AX-109997800 (5B), AX-95252437 (6D), AX-86163952 (7B), AX-110962394 (7B) (the chromosome where the locus is located is indicated in parentheses); the remaining loci are the same or similar to the reported QTL or Yr gene positions. The two loci located on chromosome 5B partially coincided with Qyr.pd-5B.1 between the markers Xbarc275 and XIWA2095, and were very close to the reported QTLs related to stripe rust disease type and QTLs associated with infection type, respectively, so it was speculated that there may be genes related to stripe rust type or reactive type or both in the vicinity of these two loci, and two loci located on chromosome 7B were close to the Yr63 gene on this chromosome, Yr63 is located at a hot spot known for against pests and diseases in plants and animals, which was enriched with multi-nucleotide-binding and leucine rich repeat (NLR) and kinase domain encoding genes ( Mackenzie et al., 2023 ), it is speculated that it is possible to discover new defense genes near this site.
5 Conclusion
In this study, 159 ICARDA wheat varieties were tested for stripe rust resistance. The majority of these lines were resistant to races CYR32 and CYR33, suggesting their potential for widespread cultivation in Qinghai Province. These findings further enrich the repository of disease-resistance genes deployed in local wheat breeding in Qinghai Province. During the association study using 55K SNP chip data and phenotypic disease resistance data from both adult and seedling stages uncovered a total of 593 loci, 589 of which were related to CYR32 and CYR33 and distributed across 21 chromosomes. Four loci were specifically related to the adult plant stage and were distributed on chromosomes 2B, 4A, and 7B. Additionally, we identified 68 multi-effect loci which were associated with disease resistance at the seedling stage. Only one stable locus was related to disease resistance at the adult-plant stage. After analysis and comparison, 60 of the 69 loci were the same or close to the reported QTL or Yr gene positions, which were likely to be the same locus, and the specific relationship needed further study; while 9 loci were relatively far apart, which can exclude the possibility of being the same locus, they may be newly discovered loci in this study and required further precise localization research. This study layed the foundation for preventing wheat stripe rust in China by serving as a theoretical reference for the utilization of novel resistance loci and by providing guidance for the discovery of new resistance genes.
Data availability statement
The data presented in the study are deposited in the Dryad Digital Repository, DOI number: 10.5061/dryad.4xgxd25ht , and the dataset has already been published.
Author contributions
ZG: Data curation, Formal analysis, Investigation, Software, Writing – original draft. XW: Data curation, Formal analysis, Investigation, Software, Writing – original draft. YL: Formal analysis, Investigation, Software, Writing – original draft. WH: Investigation, Supervision, Writing – review & editing. XZ: Conceptualization, Funding acquisition, Methodology, Project administration, Resources, Supervision, Validation, Writing – review & editing, Data curation, Investigation.
The author(s) declare financial support was received for the research, authorship, and/or publication of this article. This research was funded by the National Natural Science Foundation of China, and the project number is 32060612 which was applied for by the corresponding author Zhang.
Acknowledgments
We would like to thank Professor Han Dejun from Northwest A&F University for providing the experimental materials for this study, and to Prof. Hou and Prof. Zhang for providing project management, funding sources, and supervising the conduct of this research and the writing of the article, as well as Senior Sister Xie, Senior Sister Li and Senior Sister Wang who helped complete this paper.
Conflict of interest
The authors declare that the research was conducted in the absence of any commercial or financial relationships that could be construed as a potential conflict of interest.
Publisher’s note
All claims expressed in this article are solely those of the authors and do not necessarily represent those of their affiliated organizations, or those of the publisher, the editors and the reviewers. Any product that may be evaluated in this article, or claim that may be made by its manufacturer, is not guaranteed or endorsed by the publisher.
Supplementary material
The Supplementary Material for this article can be found online at: https://www.frontiersin.org/articles/10.3389/fpls.2024.1377253/full#supplementary-material
Alexander, D. H., Novembre, J., Lange, K. (2009). Fast model-based estimation of ancestry in unrelated individuals. Genome Res. 19, 1655–1664. doi: 10.1101/gr.094052.109
PubMed Abstract | CrossRef Full Text | Google Scholar
Bradbury, P. J., Zhang, Z., Kroon, D. E., Casstevens, T. M., Ramdoss, Y., Buckler, E. S. (2007). TASSEL: software for association mapping of complex traits in diverse samples. Bioinformatics 23, 2633–2635. doi: 10.1093/bioinformatics/btm308
Breseghello, F., Sorrells, M. E. (2006). Association mapping of kernel size and milling quality in wheat (Triticum aestivum L.) cultivars. Genetics 172, 1165–1177. doi: 10.1534/genetics.105.044586
Bulli, P., Zhang, J., Chao, S., Chen, X, Pumphrey, M. (2016). Genetic architecture of resistance to stripe rust in a global winter wheat germplasm collection. G3 (Bethesda) 6, 2237–2253. doi: 10.1534/g3.116.028407
Cao, Y. J., Xie, J. Z., Wu, Q. H., Chen, Y. X., Wang, Z. Z., Zhao, H. (2015). Genetic diversity of registered wheat varieties in Henan province based on pedigree and single-nucleotide polymorphism. Acta Agronomica Sin. 41, 197–206. doi: 10.3724/SP.J.1006.2015.00197
CrossRef Full Text | Google Scholar
Chen, W. Q., Kang, Z. S., Ma, Z. H., Xu, S. C., Jin, S. L., Jiang, Y. Y. (2013). Integrated Management of Wheat Stripe Rust Caused by Puccinia striiformis f. sp. tritici in Chin. Scientia Agricultura Sin. 46, 4254–4262. doi: 10.3864/j.issn.0578-1752.2013.20.008
Chen, X. M. (2005). Epidemiology and control of stripe rust Puccinia striiformis f. sp. tritici on wheat. Can. J. Plant Pathol. 27, 314–337. doi: 10.1080/07060660509507230
Cheng, Y. K., Yao, F. J., Ye, X. L., Jiang, Q. T., Li, W., Deng, M., et al. (2019). Construction of linkage map of the meta quantitative trait loci (MQTL) on stripe rust resistance in wheat (Triticum aestivum L.). Acta Phytopathologica Sin. 49, 632–649. doi: 10.13926/j.cnki.apps.000292
Dai, M. F., Mu, J. M., Wang, X. T., Wang, Q. L., Yu, S. Z., Huang, S., et al. (2019). Screening of stripe rust resistance molecular detection of yr genes of wheat germplasms from ICARDA. J. Triticeae Crops 39, 934–940.
Google Scholar
Danecek, P., Auton, A., Abecasis, G., Albers, C. A., Banks, E., DePristo, M. A., et al. (2011). The variant call format and VCFtools. Bioinformatics 27, 2156–2158. doi: 10.1093/bioinformatics/btr330
Dong, N., Hu, H. Y., Hu, T. Z., Li, G., Li, X. J., Chen, X. D., et al. (2019). Molecular detection and distribution of stripe rust resistance genes yr5 , yr10 and yr18 among 384 wheat germplasms. Acta Agriculturae Boreali-occidentalis Sin. 28, 1960–1968.
Feng, J., Yao, F., Wang, M., See, D. R., Chen, X. (2023). Molecular mapping of yr85 and comparison with other genes for resistance to stripe rust on wheat chromosome 1B. Plant Dis. 107, 3585–3591. doi: 10.1094/PDIS-11-22-2600-RE
Flint-Garcia, S. A., Thuillet, A. C., Yu, J., Pressoir, G., Romero, S. M., Mitchell, S. E., et al. (2005). Maize association population: a high-resolution platform for quantitative trait locus dissection. Plant J. 44, 1054–1064. doi: 10.1111/j.1365-313X.2005.02591.x
Huang, J., Jia, Q. Z., Zhang, B., Sun, Z. Y., Huang, M. M., Jin, S. L., et al. (2018). Epidemic forecasting of new strains G22-9 (CYR34) and G22-14 of Puccinia striiformis f. sp. tritici in wheat in Gansu Province. J. Plant Prot. 45, 101–108. doi: 10.13802/j.cnki.zwbhxb.2018.2018910
Juliana, P., Singh, R. P., Singh, P. K., Crossa, J., Huerta-Espino, J., Lan, C., et al. (2017). Genomic and pedigree-based prediction for leaf, stem, and stripe rust resistance in wheat. Theor. Appl. Genet. 130, 1415–1430. doi: 10.1007/s00122-017-2897-1
Klymiuk, V., Chawla, H. S., Wiebe, K., Ens, J., Fatiukha, A., Govta, L., et al. (2022). Discovery of stripe rust resistance with incomplete dominance in wild emmer wheat using bulked segregant analysis sequencing. Commun. Biol. 5, 826. doi: 10.1038/s42003-022-03773-3
Kumar, D., Chhokar, V., Sheoran, S., Singh, R., Sharma, P., Jaiswal, S., et al. (2020). Characterization of genetic diversity and population structure in wheat using array based SNP markers. Mol. Biol. Rep. 47, 293–306. doi: 10.1007/s11033-019-05132-8
Li, J., Dundas, I., Dong, C., Li, G., Trethowan, R., Yang, Z., et al. (2020). Identification and characterization of a new stripe rust resistance gene Yr83 on rye chromosome 6R in wheat. Theor. Appl. Genet. 133, 1095–1107. doi: 10.1007/s00122-020-03534-y
Li, S. S., Yi, T. F., Xu, K., Zhang, S. H., Zhao, Y., Yang, X. J. (2019). Genetic diversity of wheat varieties in hebei province based on single-nucleotide polymorphism. Mol. Plant Breed. 17, 6850–6859. doi: 10.13271/j.mpb.017.006850
Line, R. F. (2002). Stripe rust of wheat and barley in North America: a retrospective historical review. Annu. Rev. Phytopathol. 40, 75–118. doi: 10.1146/annurev.phyto.40.020102.111645
Liu, J., Feng, B., Xu, Z. B., Fan, X. L., Wang, T. (2017). “Genome-wide association study of wheat plant height traits,” in Summary Collection of the 8th Wheat Genomics and Molecular Breeding Conference. (No. 12 Zhongguancun South Street, Haidian District, Beijing: The Crop Science Society of China), 126.
Liu, K., Muse, S. V. (2005). PowerMarker: an integrated analysis environment for genetic marker analysis. Bioinformatics 21, 2128–2129. doi: 10.1093/bioinformatics/bti282
Liu, L., Wang, M. N., Feng, J. Y., See, D. R., Chao, S. M., Chen, X. M. (2018). Combination of all-stage and high-temperature adult-plant resistance QTL confers high-level, durable resistance to stripe rust in winter wheat cultivar Madsen. Theor. Appl. Genet. 131, 1835–1849. doi: 10.1007/s00122-018-3116-4
Liu, L., Wang, M., Zhang, Z., See, D. R., Chen, X. (2020). Identification of stripe rust resistance loci in U.S. Spring wheat cultivars and breeding lines using genome-wide association mapping and yr gene markers. Plant Dis. 104, 2181–2192. doi: 10.1094/PDIS-11-19-2402-RE
Liu, Y. K., Zhu, Z. W., Chen, L., Zou, J., Tong, H. W., Zhu, G., et al. (2020). Revealing the genetic diversity of wheat varieties (Lines) in China based on SNP markers. Acta Agronomica Sin. 46, 307–314. doi: 10.3724/SP.J.1006.2020.91039
Lu, J. N. (2022). Identification of the drought tolerance at seedling stage and discovery of the excellent germplasm in the ICARDA introduced wheat . (China National Knowledge Infrastructure (CNKI)).
Luo, R., Wu, W. L., Zhang, Y., Li, Y. H. (2010). SSR marker and its application to crop genetics and breeding. Genomics Appl. Biol. 29, 137–143.
Maccaferri, M., Zhang, J., Bulli, P., Abate, Z., Chao, S., Cantu, D., et al. (2015). A genome-wide association study of resistance to stripe rust (Puccinia striiformis f. sp. tritici) in a worldwide collection of hexaploid spring wheat (Triticum aestivum L.). G3 (Bethesda) 5, 449–465. doi: 10.1534/g3.114.014563
Mackenzie, A., Norman, M., Gessese, M., Chen, C., Sørensen, C., Hovmøller, M., et al. (2023). Wheat stripe rust resistance locus YR63 is a hot spot for evolution of defence genes - a pangenome discovery. BMC Plant Biol. 23, 590. doi: 10.1186/s12870-023-04576-2
Purcell, S., Neale, B., Todd-Brown, K., Thomas, L., Ferreira, M. A., Bender, D., et al. (2007). PLINK: a tool set for whole-genome association and population-based linkage analyses. Am. J. Hum. Genet. 81, 559–575. doi: 10.1086/519795
Tehseen, M. M., Istipliler, D., Kehel, Z., Sansaloni, C. P., da Silva Lopes, M., Kurtulus, E., et al. (2021). Genetic diversity and population structure analysis of triticum aestivum L. Landrace panel from Afghanistan. Genes (Basel) 12 (3), 340. doi: 10.3390/genes1203034
Thornsberry, J. M., Goodman, M. M., Doebley, J., Kresovich, S., Nielsen, D., Buckler, E. S. (2001). Dwarf8 polymorphisms associate with variation in flowering time. Nat. Genet. 28, 286–289. doi: 10.1038/90135
Wang, X., Dong, L., Hu, J., Pang, Y., Hu, L., Xiao, G. (2019). Dissecting genetic loci affecting grain morphological traits to improve grain weight via nested association mapping. Theor. Appl. Genet. 132, 3115–3128. doi: 10.1007/s00122-019-03410-4
Wang, C., Li, X., Gao, Q., Wang, B. T. (2016). The occurrence patterns and control strategies of wheat stripe rust in Ningqiang County. Shaanxi J. Agric. Sci. 62, 88–90.
Wu, J. H., Wei, R., Liu, S. J., Wang, Q. L., Nie, X. J., Song, W. N., et al. (2020). “Genome-wide association study combined with multi-environmental phenotypes for rapid identification of candidate genes for wheat stripe rust resistance,” in Summary Collection of the 19th Academic Annual Conference of the Crop Science Society of China. (No. 12 Zhongguancun South Street, Haidian District, Beijing: The Crop Science Society of China), 191.
Xu, X., Yang, X. C., Xu, R. H. (2020). Molecular detection of resistance to stripe rust in 29 wheat germplasm resources. J. Guiyang Univ. (Natural Sciences) 15, 80–83. doi: 10.16856/j.cnki.52-1142/n.2020.03.018
Yao, F. J. (2022). Identification and genome-wide association studies of stripe rust resistance in wheat germplasm . (China National Knowledge Infrastructure (CNKI)).
Yao, Q., Guo, Q. Y., Yan, J. H., Zhang, G., Hou, S. Y., Chen, W. Q. (2014). Survey on overwintering Puccinia striiformis f. sp. tritici at different altitudes in eastern Qinghai. J. Plant Prot. 41, 578–583. doi: 10.13802/j.cnki.zwbhxb.2014.05.030
Zeng, Q. D., Shen, C., Yuan, F. P., Wang, Q. L., Wu, J. H., Xue, W. B., et al. (2015). The resistance evaluation of the Yr genes to the main prevalent pathotypes of Puccini a striiformis f. sp. tririci in China. Acta Phytopathologica Sin. 45, 641–650. doi: 10.13926/j.cnki.apps.2015.06.011
Zhang, Y. B. (2022). Evaluation and genetic testing of stripe rust resistance of candidate varieties in winter wheat production areas in China. China National Knowledge Infrastructure (CNKI). doi: 10.27409/dcnki.gxbnu.2022.000214
Zhang, X. F., Chen, J. X., Liu, Y. X., Yao, Q. (2022). Stripe rust resistance evaluation and gene detection of 44 wheat varieties (Lines) in Qinghai. J. Northwest. A & F Univ. (Natural Science Edition) . 50, 11, 101–109. doi: 10.13207/j.cnki.jnwafu.2022.11.012
Zhang, X. J., Hou, W. W. (2022). Identification and molecular detection of stripe rust resistance of spring wheat in qinghai province. Mol. Plant Breed. 20, 149–154. doi: 10.13271/j.mpb.020.000149
Zhang, Z. J., Ren, Y. M., Ni, Y., Wang, D., Zhang, H. Y., Chen, J. (2019). “Evaluation of wheat resistance to stripe rust and exploration of resistance sites,” in Summary Collection of the 2019 Annual Conference of the Crop Science Society of China. (No. 12 Zhongguancun South Street, Haidian District, Beijing: The Crop Science Society of China), 319.
Zhang, D. Q., Song, X. P., Feng, J., Lian, J. F., Sun, D. J. (2016). Genetic dissection on the derived lines from wheat cultivar zhou 8425 band widely grown cultivars in Huang-huai region. J. Triticeae Crops 36, 1328–1334.
Zhang, G. S., Zhao, Y. Y., Kang, Z. S., Zhao, J. (2020). First Report of a Puccinia striiformis f. sp. tritici Race Virulent to Wheat Stripe Rust Resistance Gene Yr5 in China. Plant Dis. 104, 284. doi: 10.1094/pdis-05-19-0901-pdn
Zhu, Z., Cao, Q., Han, D., Wu, J., Wu, L., Tong, J., et al. (2023). Molecular characterization and validation of adult-plant stripe rust resistance gene Yr86 in Chinese wheat cultivar Zhongmai 895. Theor. Appl. Genet. 136, 142. doi: 10.1007/s00122-023-04374-2
Keywords: ICARDA, wheat varieties, stripe rust, 55K SNP, genome-wide association study
Citation: Gao Z, Wang X, Li Y, Hou W and Zhang X (2024) Evaluation of stripe rust resistance and genome-wide association study in wheat varieties derived from the International Center for Agricultural Research in the Dry Areas. Front. Plant Sci. 15:1377253. doi: 10.3389/fpls.2024.1377253
Received: 27 January 2024; Accepted: 21 March 2024; Published: 09 April 2024.
Reviewed by:
Copyright © 2024 Gao, Wang, Li, Hou and Zhang. This is an open-access article distributed under the terms of the Creative Commons Attribution License (CC BY) . The use, distribution or reproduction in other forums is permitted, provided the original author(s) and the copyright owner(s) are credited and that the original publication in this journal is cited, in accordance with accepted academic practice. No use, distribution or reproduction is permitted which does not comply with these terms.
*Correspondence: Xiaojuan Zhang, [email protected]
† These authors have contributed equally to this work and share first authorship
Disclaimer: All claims expressed in this article are solely those of the authors and do not necessarily represent those of their affiliated organizations, or those of the publisher, the editors and the reviewers. Any product that may be evaluated in this article or claim that may be made by its manufacturer is not guaranteed or endorsed by the publisher.
Thank you for visiting nature.com. You are using a browser version with limited support for CSS. To obtain the best experience, we recommend you use a more up to date browser (or turn off compatibility mode in Internet Explorer). In the meantime, to ensure continued support, we are displaying the site without styles and JavaScript.
- View all journals
- Explore content
- About the journal
- Publish with us
- Sign up for alerts
- Review Article
- Published: 09 April 2024
Plant responses to changing rainfall frequency and intensity
- Andrew F. Feldman ORCID: orcid.org/0000-0003-1547-6995 1 , 2 ,
- Xue Feng ORCID: orcid.org/0000-0003-1381-3118 3 , 4 ,
- Andrew J. Felton 5 ,
- Alexandra G. Konings ORCID: orcid.org/0000-0002-2810-1722 6 ,
- Alan K. Knapp ORCID: orcid.org/0000-0003-1695-4696 7 ,
- Joel A. Biederman 8 &
- Benjamin Poulter ORCID: orcid.org/0000-0002-9493-8600 1
Nature Reviews Earth & Environment volume 5 , pages 276–294 ( 2024 ) Cite this article
88 Accesses
20 Altmetric
Metrics details
- Carbon cycle
- Climate-change ecology
Regardless of annual rainfall amount changes, daily rainfall events are becoming more intense but less frequent with anthropogenic warming. Larger rainfall events and longer dry spells have complex and sometimes opposing effects on plant photosynthesis and growth, challenging abilities to understand broader consequences on the carbon cycle. In this Review, we evaluate global plant responses to rainfall regimes characterized by fewer, larger rainfall events across evidence from field experiments, satellites and models. Plant function responses vary between −28% and 29% (5th to 95th percentile) under fewer, larger rainfall events, with the direction of response contingent on climate; productivity increases are more common in dry ecosystems (46% positive; 20% negative), whereas responses are typically negative in wet ecosystems (28% positive; 51% negative). Contrasting responses in dry and wet ecosystems are attributed to nonlinear plant responses to soil moisture driven by several ecohydrological mechanisms. For example, dry ecosystem plants are more sensitive to large rainfall pulses compared with wet ecosystem plants, partly driving dry ecosystem positive responses to fewer, larger rainfall events. Knowledge gaps remain over optimal rainfall frequencies for photosynthesis, the relative dominance of rainfall pulse and dry spell mechanisms and the disproportionate role of extreme rainfall pulses on plant function.
This is a preview of subscription content, access via your institution
Access options
Access Nature and 54 other Nature Portfolio journals
Get Nature+, our best-value online-access subscription
24,99 € / 30 days
cancel any time
Subscribe to this journal
Receive 12 digital issues and online access to articles
92,52 € per year
only 7,71 € per issue
Rent or buy this article
Prices vary by article type
Prices may be subject to local taxes which are calculated during checkout
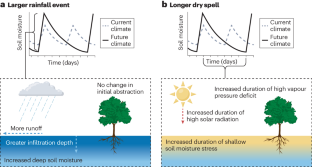
Similar content being viewed by others
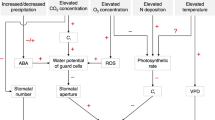
Stomatal responses of terrestrial plants to global change
Xingyun Liang, Defu Wang, … David S. Ellsworth
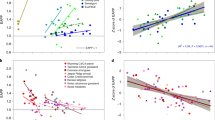
Contrasting responses of woody and grassland ecosystems to increased CO2 as water supply varies
Yude Pan, Robert B. Jackson, … Yiqi Luo
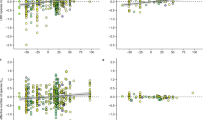
Responses of plant diversity to precipitation change are strongest at local spatial scales and in drylands
Lotte Korell, Harald Auge, … Tiffany M. Knight
Data availability
CMIP6 rainfall projections can be obtained from https://cds.climate.copernicus.eu . Observation-based rainfall data sets from MERRA, CPC and GPCC can be obtained from https://gmao.gsfc.nasa.gov/reanalysis/MERRA-2/data_access , https://psl.noaa.gov/data/gridded/data.cpc.globalprecip.html and https://psl.noaa.gov/data/gridded/data.gpcc.html , respectively. FLUXNET observations can be downloaded from https://fluxnet.org .
Ruehr, S. et al. Evidence and attribution of the enhanced land carbon sink. Nat. Rev. Earth Environ. 4 , 518–534 (2023).
Article CAS Google Scholar
Friedlingstein, P. et al. Global Carbon Budget 2022. Earth Syst. Sci. Data 14 , 4811–4900 (2022).
Article Google Scholar
Yang, Y. et al. Evapotranspiration on a greening Earth. Nat. Rev. Earth Environ. 4 , 626–641 (2023).
Green, J. K. et al. Regionally strong feedbacks between the atmosphere and terrestrial biosphere. Nat. Geosci. 10 , 410–414 (2017).
Forzieri, G., Alkama, R., Miralles, D. G. & Cescatti, A. Satellites reveal contrasting responses of regional climate to the widespread greening of Earth. Science 360 , 1180–1184 (2018).
Spracklen, D. V., Arnold, S. R. & Taylor, C. M. Observations of increased tropical rainfall preceded by air passage over forests. Nature 489 , 282–285 (2012).
Nemani, R. R. et al. Climate-driven increases in global terrestrial net primary production from 1982 to 1999. Science 300 , 1560–1563 (2003).
Humphrey, V. et al. Sensitivity of atmospheric CO 2 growth rate to observed changes in terrestrial water storage. Nature 560 , 628–631 (2018).
Madani, N., Kimball, J. S., Jones, L. A., Parazoo, N. C. & Guan, K. Global Analysis of bioclimatic controls on ecosystem productivity using satellite observations of solar-induced chlorophyll fluorescence. Remote Sens. 9 , 530 (2017).
Poulter, B. et al. Contribution of semi-arid ecosystems to interannual variability of the global carbon cycle. Nature 509 , 600–603 (2014).
Ahlström, A. et al. The dominant role of semi-arid ecosystems in the trend and variability of the land CO 2 sink. Science 348 , 895–900 (2015).
Green, J. K. et al. Large influence of soil moisture on long-term terrestrial carbon uptake. Nature 565 , 476–479 (2019).
Reichstein, M. et al. Climate extremes and the carbon cycle. Nature 500 , 287–295 (2013).
Ciais, P. et al. Europe-wide reduction in primary productivity caused by the heat and drought in 2003. Nature 437 , 529–533 (2005).
Reyer, C. P. O. et al. A plant’s perspective of extremes: terrestrial plant responses to changing climatic variability. Glob. Chang. Biol. 19 , 75–89 (2013).
Sala, O. E., Parton, W. J., Joyce, L. A. & Lauenroth, W. K. Primary production of the central grassland region of the United States. Ecology 69 , 40–45 (1988).
Biederman, J. A. et al. CO 2 exchange and evapotranspiration across dryland ecosystems of southwestern North America. Glob. Chang. Biol. 23 , 4204–4221 (2017).
Ukkola, A. M. et al. Annual precipitation explains variability in dryland vegetation greenness globally but not locally. Glob. Chang. Biol. 27 , 4367–4380 (2021).
Pendergrass, A. G. & Knutti, R. The uneven nature of daily precipitation and its change. Geophys. Res. Lett. 45 , 11,980–11,988 (2018).
Fowler, H. J. et al. Anthropogenic intensification of short-duration rainfall extremes. Nat. Rev. Earth Environ. 2 , 107–122 (2021).
Kannenberg, S. A., Bowling, D. R. & Anderegg, W. R. L. Hot moments in ecosystem fluxes: high GPP anomalies exert outsized influence on the carbon cycle and are differentially driven by moisture availability across biomes. Environ. Res. Lett. 15 , 054004 (2020).
Trenberth, K. E. Changes in precipitation with climate change. Clim. Res. 47 , 123–138 (2011).
Pendergrass, A. G., Knutti, R., Lehner, F., Deser, C. & Sanderson, B. M. Precipitation variability increases in a warmer climate. Sci. Rep. 7 , 1–9 (2017). This study estimates daily rainfall frequency and intensity trends, both observed and model projected, and compares with trends with annual rainfall totals.
Giorgi, F., Raffaele, F. & Coppola, E. The response of precipitation characteristics to global warming from climate projections. Earth Syst. Dyn. 10 , 73–89 (2019). An investigation of daily rainfall frequency and intensity-projected trends within CMIP5 experiments.
Holdrege, M. C., Kulmatiski, A., Palmquist, K. A. & Beard, K. H. Precipitation intensification increases shrub dominance in arid, not mesic, ecosyst. Ecosystems 26 , 568–584 (2023).
Thomey, M. L. et al. Effect of precipitation variability on net primary production and soil respiration in a Chihuahuan Desert grassland. Glob. Chang. Biol. 17 , 1505–1515 (2011).
Short Gianotti, D. J., Akbar, R., Feldman, A. F., Salvucci, G. D. & Entekhabi, D. Terrestrial evaporation and moisture drainage in a warmer climate. Geophys. Res. Lett. 47 , e2019GL086498 (2020).
McColl, K. A. et al. Global characterization of surface soil moisture drydowns. Geophys. Res. Lett. 44 , 3682–3690 (2017).
Zhang, F., Biederman, J. A. & Dannenberg, M. P. Five decades of observed daily precipitation reveal longer and more variable drought events across much of the Western United States. Geophys. Res. Lett. 48 , e2020GL092293 (2021).
Feldman, A. F., Short Gianotti, D. J., Trigo, I. F., Salvucci, G. D. & Entekhabi, D. Land–atmosphere drivers of landscape-scale plant water content loss. Geophys. Res. Lett. 47 , e2020GL090331 (2020).
Grossiord, C. et al. Plant responses to rising vapor pressure deficit. N. Phytol. 226 , 1550–1566 (2020).
Ross, I. et al. How do variations in the temporal distribution of rainfall events affect ecosystem fluxes in seasonally water-limited Northern Hemisphere shrublands and forests? Biogeosciences 9 , 1007–1024 (2012).
Liu, J. et al. Impact of temporal precipitation variability on ecosystem productivity. Wiley Interdiscip. Rev. Water 7 , e1481 (2020).
Knapp, A. K. et al. Rainfall variability, carbon cycling, and plant species diversity in a mesic grassland. Science 298 , 2202–2205 (2002). One of the first field experiments evaluating effects of fewer, larger rainfall events on soil and plants at a Kansas site where many rainfall manipulation experiments have been performed.
Liu, W. J. et al. Repackaging precipitation into fewer, larger storms reduces ecosystem exchanges of CO 2 and H 2 O in a semiarid steppe. Agric. For. Meteorol. 247 , 356–364 (2017).
Fay, P. A., Kaufman, D. M., Nippert, J. B., Carlisle, J. D. & Harper, C. W. Changes in grassland ecosystem function due to extreme rainfall events: implications for responses to climate change. Glob. Chang. Biol. 14 , 1600–1608 (2008).
Heisler-White, J. L., Blair, J. M., Kelly, E. F., Harmoney, K. & Knapp, A. K. Contingent productivity responses to more extreme rainfall regimes across a grassland biome. Glob. Chang. Biol. 15 , 2894–2904 (2009).
Piao, S. et al. Characteristics, drivers and feedbacks of global greening. Nat. Rev. Earth Environ. 1 , 14–27 (2020).
Baudena, M., Boni, G., Ferraris, L., von Hardenberg, J. & Provenzale, A. Vegetation response to rainfall intermittency in drylands: results from a simple ecohydrological box model. Adv. Water Resour. 30 , 1320–1328 (2007).
Feng, X., Porporato, A. & Rodriguez-Iturbe, I. Changes in rainfall seasonality in the tropics. Nat. Clim. Chang. 3 , 811–815 (2013).
Su, J., Zhang, Y. & Xu, F. Divergent responses of grassland productivity and plant diversity to intra- annual precipitation variability across climate regions: a global synthesis. J. Ecol. 111 , 1–14 (2023). Synthesized plant responses to changing rainfall frequency and intensity from field experiments across the globe.
Knapp, A. K. et al. Consequences of more extreme precipitation regimes for terrestrial ecosystems. Bioscience 58 , 811–821 (2008). Uses a soil water bucket model to provide a framework for why plants in dry and wet ecosystems would have opposing responses to fewer, larger wet days.
Wang, L. et al. Dryland productivity under a changing climate. Nat. Clim. Chang. 12 , 981–994 (2022).
Lian, X. et al. Multifaceted characteristics of dryland aridity changes in a warming world. Nat. Rev. Earth Environ. 2 , 232–250 (2021).
Huxman, T. E. et al. Precipitation pulses and carbon fluxes in semiarid and arid ecosystems. Oecologia 141 , 254–268 (2004).
Held, I. M. & Soden, B. J. Robust responses of the hydrological cycle to global warming. J. Clim. 19 , 5686–5699 (2006).
Lee, J.-Y. et al. in Climate Change 2021 — The Physical Science Basis: Contribution of Working Group I to the Sixth Assessment Report of the Intergovernmental Panel on Climate Change Ch. 4 (eds Masson-Delmotte, V. et al.) 553–672 (Cambridge Univ. Press, 2023).
Byrne, M. P. & O’Gorman, P. A. The response of precipitation minus evapotranspiration to climate warming: why the ‘wet-get-wetter, dry-get-drier’ scaling does not hold over land. J. Clim. 28 , 8078–8093 (2015).
Zaitchik, B. F., Rodell, M., Biasutti, M. & Seneviratne, S. I. Wetting and drying trends under climate change. Nat. Water 1 , 502–513 (2023).
Alexander, L. V. et al. Global observed changes in daily climate extremes of temperature and precipitation. J. Geophys. Res. Atmos. 111 , 1–22 (2006).
Ziese, M. et al. GPCC full data daily version 2022 at 1.0°: daily land-surface precipitation from rain-gauges built on GTS-based and historic data. GPCC https://doi.org/10.5676/DWD_GPCC/FD_D_V2022_100 (2022).
Reichle, R. H. et al. Land surface precipitation in MERRA-2. J. Clim. 30 , 1643–1664 (2017).
Chen, M. et al. Assessing objective techniques for gauge-based analyses of global daily precipitation. J. Geophys. Res. Atmos. 113 , 1–13 (2008).
Google Scholar
Pendergrass, A. G. What precipitation is extreme? Science 360 , 1072–1073 (2018).
O’Gorman, P. A. & Schneider, T. The physical basis for increases in precipitation extremes in simulations of 21st-century climate change. Proc. Natl Acad. Sci. USA 106 , 14773–14777 (2009).
Pendergrass, A. G. & Gerber, E. P. The rain is askew: two idealized models relating vertical velocity and precipitation distributions in a warming world. J. Clim. 29 , 6445–6462 (2016).
Pfahl, S., O’Gorman, P. A. & Fischer, E. M. Understanding the regional pattern of projected future changes in extreme precipitation. Nat. Clim. Chang. 7 , 423–427 (2017).
Lu, J., Vecchi, G. A. & Reichler, T. Expansion of the Hadley cell under global warming. Geophys. Res. Lett. 34 , 2–6 (2007).
Vecchi, G. A. & Soden, B. J. Global warming and the weakening of the tropical circulation. J. Clim. 20 , 4316–4340 (2007).
Donat, M. G., Lowry, A. L., Alexander, L. V., O’Gorman, P. A. & Maher, N. More extreme precipitation in the world’s dry and wet regions. Nat. Clim. Chang. 6 , 508–513 (2016).
Donat, M. G., Angélil, O. & Ukkola, A. M. Intensification of precipitation extremes in the world’s humid and water-limited regions. Environ. Res. Lett. 14 , 065003 (2019).
Fischer, E. M., Beyerle, U. & Knutti, R. Robust spatially aggregated projections of climate extremes. Nat. Clim. Chang. 3 , 1033–1038 (2013).
Miranda, J., Padilla, F. M., Lázaro, R. & Pugnaire, F. I. Do changes in rainfall patterns affect semiarid annual plant communities? J. Veg. Sci. 20 , 269–276 (2009).
Zhang, J. et al. Moderately prolonged dry intervals between precipitation events promote production in Leymus chinensis in a semi-arid grassland of Northeast China. BMC Plant. Biol. 21 , 1–11 (2021).
Grant, K., Kreyling, J., Beierkuhnlein, C. & Jentsch, A. Importance of seasonality for the response of a mesic temperate grassland to increased precipitation variability and warming. Ecosystems 20 , 1454–1467 (2017).
Ma, Y., Zhang, T. & Liu, X. Effect of intensity of small rainfall simulation in spring on annuals in Horqin Sandy Land, China. Environ. Earth Sci. 74 , 727–735 (2015).
Padilla, F. M. et al. Effects of extreme rainfall events are independent of plant species richness in an experimental grassland community. Oecologia 191 , 177–190 (2019).
Schuster, M. J., Smith, N. G. & Dukes, J. S. Responses of aboveground C and N pools to rainfall variability and nitrogen deposition are mediated by seasonal precipitation and plant community dynamics. Biogeochemistry 129 , 389–400 (2016).
Arca, V., Power, S. A., Delgado-Baquerizo, M., Pendall, E. & Ochoa-Hueso, R. Seasonal effects of altered precipitation regimes on ecosystem-level CO 2 fluxes and their drivers in a grassland from Eastern Australia. Plant Soil 460 , 435–451 (2021).
Zhang, F. et al. Precipitation temporal repackaging into fewer, larger storms delayed seasonal timing of peak photosynthesis in a semi‐arid grassland. Funct. Ecol. 36 , 646–658 (2021).
Holdrege, M. C., Beard, K. H. & Kulmatiski, A. Woody plant growth increases with precipitation intensity in a cold semiarid system. Ecology 102 , 1–11 (2021).
Fay, P. A. et al. Relative effects of precipitation variability and warming on tallgrass prairie ecosystem function. Biogeosciences 8 , 3053–3068 (2011).
Kulmatiski, A. & Beard, K. H. Woody plant encroachment facilitated by increased precipitation intensity. Nat. Clim. Chang. 3 , 833–837 (2013).
Berry, R. S. & Kulmatiski, A. A savanna response to precipitation intensity. PLoS ONE 12 , 1–18 (2017).
Williams, K. J., Wilsey, B. J., McNaughton, S. J. & Banyikwa, F. F. Temporally variable rainfall does not limit yields of Serengeti grasses. Oikos 81 , 463 (1998).
Xie, Y., Li, Y., Xie, T., Meng, R. & Zhao, Z. Impact of artificially simulated precipitation pattern change on the growth and morphology of Reaumuria soongarica seedlings in Hexi Corridor of China. Sustain 12 , 2439 (2020).
Good, S. P. & Caylor, K. K. Climatological determinants of woody cover in Africa. Proc. Natl Acad. Sci. USA 108 , 4902–4907 (2011).
Zhao, H., Jia, G., Xu, X., Zhang, A. & Wang, H. Divergent effects of intensified precipitation on primary production in global drylands. Sci. Total Environ. 892 , 164736 (2023).
Case, M. F. & Staver, A. C. Soil texture mediates tree responses to rainfall intensity in African savannas. N. Phytol. 219 , 1363–1372 (2018).
Ritter, F., Berkelhammer, M. & Garcia-Eidell, C. Distinct response of gross primary productivity in five terrestrial biomes to precipitation variability. Commun. Earth Environ. 1 , 34 (2020).
Fang, J. et al. Precipitation patterns alter growth of temperate vegetation. Geophys. Res. Lett. 32 , 1–5 (2005).
Xu, X. et al. Tree cover shows strong sensitivity to precipitation variability across the global tropics. Glob. Ecol. Biogeogr. 27 , 450–460 (2018).
Zhang, Y. et al. Extreme precipitation patterns and reductions of terrestrial ecosystem production across biomes. J. Geophys. Res. Biogeosci. 118 , 148–157 (2013).
Zhang, W., Brandt, M., Tong, X., Tian, Q. & Fensholt, R. Impacts of the seasonal distribution of rainfall on vegetation productivity across the Sahel. Biogeosciences 15 , 319–330 (2018).
Guo, Q. et al. Contrasting responses of gross primary productivity to precipitation events in a water-limited and a temperature-limited grassland ecosystem. Agric. For. Meteorol. 214–215 , 169–177 (2015).
D’Onofrio, D., Sweeney, L., von Hardenberg, J. & Baudena, M. Grass and tree cover responses to intra-seasonal rainfall variability vary along a rainfall gradient in African tropical grassy biomes. Sci. Rep. 9 , 1–10 (2019).
Porporato, A., Daly, E. & Rodriguez-Iturbe, I. Soil water balance and ecosystem response to climate change. Am. Nat. 164 , 625–632 (2004). This study uses a minimalist process model to predict and attribute plant responses to fewer, larger rainfall events at the Knapp et al. (2002) Kansas field experiment.
Zhang, D. H., Li, X. R., Zhang, F., Zhang, Z. S. & Chen, Y. L. Effects of rainfall intensity and intermittency on woody vegetation cover and deep soil moisture in dryland ecosystems. J. Hydrol. 543 , 270–282 (2016).
Nordbotten, J. M., Rodriguez-Iturbe, I. & Celia, M. A. Stochastic coupling of rainfall and biomass dynamics. Water Resour. Res. 43 , 1–7 (2007).
Xu, X., Medvigy, D. & Rodriguez-Iturbe, I. Relation between rainfall intensity and savanna tree abundance explained by water use strategies. Proc. Natl Acad. Sci. USA 112 , 12992–12996 (2015).
Guan, K. et al. Continental-scale impacts of intra-seasonal rainfall variability on simulated ecosystem responses in Africa. Biogeosciences 11 , 6939–6954 (2014).
Medvigy, D., Wofsy, S. C., Munger, J. W. & Moorcroft, P. R. Responses of terrestrial ecosystems and carbon budgets to current and future environmental variability. Proc. Natl Acad. Sci. USA 107 , 8275–8280 (2010).
Peng, S. et al. Precipitation amount, seasonality and frequency regulate carbon cycling of a semi-arid grassland ecosystem in Inner Mongolia, China: a modeling analysis. Agric. For. Meteorol. 178–179 , 46–55 (2013).
Fay, P. A. et al. Altered rainfall patterns, gas exchange, and growth in grasses and forbs. Int. J. Plant Sci. 163 , 549–557 (2002).
Griffin-Nolan, R. J., Slette, I. J. & Knapp, A. K. Deconstructing precipitation variability: rainfall event size and timing uniquely alter ecosystem dynamics. J. Ecol. 109 , 3356–3369 (2021).
Rodríguez-Iturbe, I., D’Odorico, P., Porporato, A. & Ridolfi, L. On the spatial and temporal links between vegetation, climate, and soil moisture. Water Resour. 35 , 3709–3722 (1999).
Laio, F., Porporato, A., Fernandez-Illescas, C. P. & Rodriguez-Iturbe, I. Plants in water-controlled ecosystems: active role in hydrologic processes and response to water stress II. Probabilistic soil moisture dynamics. Adv. Water Resour. 24 , 707–723 (2001).
Daly, E., Porporato, A. & Rodriguez-Iturbe, I. Coupled dynamics of photosynthesis, transpiration, and soil water balance. Part II: stochastic analysis and ecohydrological significance. J. Hydrometeorol. 5 , 559–566 (2004).
Daly, E., Porporato, A. & Rodríguez-Iturbe, I. Coupled dynamics of photosynthesis, transpiration, and soil water balance. Part I: upscaling from hourly to daily level. J. Hydrometeorol. 5 , 546–558 (2004).
Seneviratne, S. I. et al. Investigating soil moisture–climate interactions in a changing climate: a review. Earth Sci. Rev. 99 , 125–161 (2010).
Porporato, A., Laio, F., Ridolfi, L. & Rodriguez-Iturbe, I. Plants in water-controlled ecosystems: active role in hydrologic processes and response to water stress III. Vegetation water stress. Adv. Water Resour. 24 , 725–744 (2001).
Guan, K. et al. Simulated sensitivity of African terrestrial ecosystem photosynthesis to rainfall frequency, intensity, and rainy season length. Environ. Res. Lett. 13 , 025013 (2018).
Nippert, J. B., Knapp, A. K. & Briggs, J. M. Intra-annual rainfall variability and grassland productivity: can the past predict the future? Plant Ecol. 184 , 65–74 (2006).
Noy-Meir, I. Desert ecosystems: environment and producers. Annu. Rev. Ecol. Syst. 4 , 25–52 (1973). This paper poses the ‘ pulse reserve paradigm ’ to describe dryland plant responses to individual rainfall events.
Felton, A. J., Slette, I. J., Smith, M. D. & Knapp, A. K. Precipitation amount and event size interact to reduce ecosystem functioning during dry years in a mesic grassland. Glob. Chang. Biol. 26 , 658–668 (2020).
Fay, P. A., Carlisle, J. D., Knapp, A. K., Blair, J. M. & Collins, S. L. Productivity responses to altered rainfall patterns in a C 4-dominated grassland. Oecologia 137 , 245–251 (2003).
Post, A. K. & Knapp, A. K. How big is big enough? Surprising responses of a semiarid grassland to increasing deluge size. Glob. Chang. Biol. 27 , 1157–1169 (2021).
Robinson, T. M. P. & Gross, K. L. The impact of altered precipitation variability on annual weed species. Am. J. Bot. 97 , 1625–1629 (2010).
Wilcox, K. R., von Fischer, J. C., Muscha, J. M., Petersen, M. K. & Knapp, A. K. Contrasting above- and belowground sensitivity of three Great Plains grasslands to altered rainfall regimes. Glob. Chang. Biol. 21 , 335–344 (2015).
Slette, I. J., Blair, J. M., Fay, P. A., Smith, M. D. & Knapp, A. K. Effects of compounded precipitation pattern intensification and drought occur belowground in a mesic grassland. Ecosystems 25 , 1265–1278 (2021).
Zeppel, M. J. B., Wilks, J. V. & Lewis, J. D. Impacts of extreme precipitation and seasonal changes in precipitation on plants. Biogeosciences 11 , 3083–3093 (2014).
Huang, H., Calabrese, S. & Rodriguez-Iturbe, I. J. Variability of ecosystem carbon source from microbial respiration is controlled by rainfall dynamics. Proc. Natl Acad. Sci. USA 118 , e2115283118 (2021).
Berg, A., Sultan, B. & De Noblet-Ducoudré, N. What are the dominant features of rainfall leading to realistic large-scale crop yield simulations in West Africa? Geophys. Res. Lett. 37 , 1–6 (2010).
Harper, C. W., Blair, J. M., Fay, P. A., Knapp, A. K. & Carlisle, J. D. Increased rainfall variability and reduced rainfall amount decreases soil CO 2 flux in a grassland ecosystem. Glob. Chang. Biol. 11 , 322–334 (2005).
Felton, A. J. et al. Climate disequilibrium dominates uncertainty in long-term projections of primary productivity. Ecol. Lett. 25 , 2688–2698 (2022).
Knapp, A. K. et al. Past, present, and future roles of long-term experiments in the LTER network. Bioscience 62 , 377–389 (2012).
Beier, C. et al. Precipitation manipulation experiments — challenges and recommendations for the future. Ecol. Lett. 15 , 899–911 (2012).
Adler, P. B., White, E. P. & Cortez, M. H. Matching the forecast horizon with the relevant spatial and temporal processes and data sources. Ecography 43 , 1729–1739 (2020).
Smith, M. D., Knapp, A. K. & Collins, S. L. A framework for assessing ecosystem dynamics in response to chronic resource alterations induced by global change. Ecology 90 , 3279–3289 (2009).
Feng, X., Dawson, T. E., Ackerly, D. D., Santiago, L. S. & Thompson, S. E. Reconciling seasonal hydraulic risk and plant water use through probabilistic soil–plant dynamics. Glob. Chang. Biol. 23 , 3758–3769 (2017).
Throop, H. L., Reichmann, L. G., Sala, O. E. & Archer, S. R. Response of dominant grass and shrub species to water manipulation: an ecophysiological basis for shrub invasion in a Chihuahuan Desert Grassland. Oecologia 169 , 373–383 (2012).
Javadian, M. et al. Thermography captures the differential sensitivity of dryland functional types to changes in rainfall event timing and magnitude. N. Phytol. 240 , 114–126 (2023).
Reynaert, S. et al. Risk of short-term biodiversity loss under more persistent precipitation regimes. Glob. Chang. Biol. 27 , 1614–1626 (2021).
Jackson, R. B., Banner, J. L., Jobbagy, E. G., Pockman, W. T. & Wall, D. H. Ecosystem carbon loss with woody plant invasion of grasslands. Nature 418 , 623–626 (2002).
Gherardi, L. A. & Sala, O. E. Enhanced precipitation variability decreases grass- and increases shrub-productivity. Proc. Natl Acad. Sci. USA 112 , 12735–12740 (2015).
Schreiner-McGraw, A. P. et al. Woody plant encroachment has a larger impact than climate change on dryland water budgets. Sci. Rep. 10 , 1–9 (2020).
Hao, Y. et al. Climate-induced abrupt shifts in structural states trigger delayed transitions in functional states. Ecol. Indic. 115 , 106468 (2020).
Harrison, S. P., Gornish, E. S. & Copeland, S. Climate-driven diversity loss in a grassland community. Proc. Natl Acad. Sci. USA 112 , 8672–8677 (2015).
Barbeta, A., Ogaya, R. & Peñuelas, J. Dampening effects of long-term experimental drought on growth and mortality rates of a Holm oak forest. Glob. Chang. Biol. 19 , 3133–3144 (2013).
Jones, S. K., Collins, S. L., Blair, J. M., Smith, M. D. & Knapp, A. K. Altered rainfall patterns increase forb abundance and richness in native tallgrass prairie. Sci. Rep. 6 , 1–10 (2016).
Zhang, Y., Keenan, T. F. & Zhou, S. Exacerbated drought impacts on global ecosystems due to structural overshoot. Nat. Ecol. Evol. 5 , 1490–1498 (2021).
Jump, A. S. et al. Structural overshoot of tree growth with climate variability and the global spectrum of drought-induced forest dieback. Glob. Chang. Biol. 23 , 3742–3757 (2017).
Collins, S. L. et al. Stability of tallgrass prairie during a 19-year increase in growing season precipitation. Funct. Ecol. 26 , 1450–1459 (2012).
Keenan, T. F. et al. Increase in forest water-use efficiency as atmospheric carbon dioxide concentrations rise. Nature 499 , 324–327 (2013).
Donohue, R. J., Roderick, M. L., McVicar, T. R. & Farquhar, G. D. Impact of CO 2 fertilization on maximum foliage cover across the globe’s warm, arid environments. Geophys. Res. Lett. 40 , 3031–3035 (2013).
Zhang, Y. et al. Increasing sensitivity of dryland vegetation greenness to precipitation due to rising atmospheric CO 2 . Nat. Commun. 13 , 4875 (2022).
Roby, M. C., Scott, R. L. & Moore, D. J. P. High vapor pressure deficit decreases the productivity and water use efficiency of rain-induced pulses in semiarid ecosystems. J. Geophys. Res. Biogeosci. 125 , 1–14 (2020).
Katul, G. G., Oren, R., Manzoni, S., Higgins, C. & Parlange, M. B. Evapotranspiration: a process driving mass transport and energy exchange in the soil–plant–atmosphere–climate system. Rev. Geophys. 50 , 1–25 (2012).
Reynolds, J. F., Kemp, P. R., Ogle, K. & Fernández, R. J. Modifying the ‘pulse-reserve’ paradigm for deserts of North America: precipitation pulses, soil water, and plant responses. Oecologia 141 , 194–210 (2004).
Fensham, R. J., Butler, D. W. & Foley, J. How does clay constrain woody biomass in drylands? Glob. Ecol. Biogeogr. 24 , 950–958 (2015).
Wei, L. et al. Experimental investigation of relationship between infiltration rate and soil moisture under rainfall conditions. Water 14 , 1–11 (2022).
Penna, D., Tromp-Van Meerveld, H. J., Gobbi, A., Borga, M. & Dalla Fontana, G. The influence of soil moisture on threshold runoff generation processes in an alpine headwater catchment. Hydrol. Earth Syst. Sci. 15 , 689–702 (2011).
Western, A. W. & Grayson, R. B. The Terrawarra data set: soil moisture patterns, soil characteristics, and hydrological flux measurements. Water Resour. Res. 34 , 2765–2768 (1998).
Vereecken, H. et al. Soil hydrology in the Earth system. Nat. Rev. Earth Environ. 3 , 573–587 (2022).
Lian, X., Zhao, W. & Gentine, P. Recent global decline in rainfall interception loss due to altered rainfall regimes. Nat. Commun. 13 , 7642 (2022).
Thomey, M. L., Collins, S. L., Friggens, M. T., Brown, R. F. & Pockman, W. T. Effects of monsoon precipitation variability on the physiological response of two dominant C 4 grasses across a semiarid ecotone. Oecologia 176 , 751–762 (2014).
Lauenroth, W. K. & Bradford, J. B. Ecohydrology of dry regions of the United States: water balance consequences of small precipitation events. Ecohydrology 5 , 46–53 (2012).
Demaria, E. M. C., Hazenberg, P. & Meles, M. B. Intensification of the North American Monsoon rainfall as observed from a long‐term high‐density gauge network geophysical research letters. Geophys. Res. Lett. 46 , 6839–6847 (2019).
Pockman, W. T. & Small, E. E. The influence of spatial patterns of soil moisture on the grass and shrub responses to a summer rainstorm in a Chihuahuan desert ecotone. Ecosystems 13 , 511–525 (2010).
McDonald, A. K., Kinucan, R. J. & Loomis, L. E. Ecohydrological interactions within banded vegetation in the northeastern Chihuahuan Desert, USA. Ecohydrology 2 , 66–71 (2009).
Schreiner-Mcgraw, A. P., Ajami, H. & Vivoni, E. R. Extreme weather events and transmission losses in arid streams. Environ. Res. Lett . 14 , 084002 (2019).
CAS Google Scholar
Scott, R. L. & Biederman, J. A. Critical zone water balance over 13 years in a semiarid savanna. Water Resour. Res. 55 , 574–588 (2019).
Nippert, J. B. & Holdo, R. M. Challenging the maximum rooting depth paradigm in grasslands and savannas. Funct. Ecol. 29 , 739–745 (2015).
Potts, D. L. et al. Antecedent moisture and seasonal precipitation influence the response of canopy-scale carbon and water exchange to rainfall pulses in a semi-arid grassland. N. Phytol. 170 , 849–860 (2006).
He, Z., Zhao, W., Liu, H. & Chang, X. The response of soil moisture to rainfall event size in subalpine grassland and meadows in a semi-arid mountain range: a case study in northwestern China’s Qilian Mountains. J. Hydrol. 420–421 , 183–190 (2012).
Tumber-Dávila, S. J., Schenk, H. J., Du, E. & Jackson, R. B. Plant sizes and shapes above and belowground and their interactions with climate. N. Phytol. 235 , 1032–1056 (2022).
Jackson, R. B. et al. A global analysis of root distributions for terrestrial biomes. Oecologia 108 , 389–411 (1996).
Raz-Yaseef, N., Yakir, D., Rotenberg, E., Schiller, G. & Cohen, S. Ecohydrology of a semi-arid forest: partitioning among water balance components and its implications for predicted precipitation changes. Ecohydrology 3 , 143–154 (2010).
Fravolini, A. et al. Precipitation pulse use by an invasive woody legume: the role of soil texture and pulse size. Oecologia 144 , 618–627 (2005).
Zhu, X. et al. Soil coarsening alleviates precipitation constraint on vegetation growth in global drylands. Environ. Res. Lett. 17 , 11 (2022).
Ogle, K., Wolpert, R. L. & Reynolds, J. F. Reconstructing plant root area and water uptake profiles. Ecology 85 , 1967–1978 (2004).
Schwinning, S., Sala, O. E., Loik, M. E. & Ehleringer, J. R. Thresholds, memory, and seasonality: understanding pulse dynamics in arid/semi-arid ecosystems. Oecologia 141 , 191–193 (2004).
Brodribb, T. J. & Cochard, H. Hydraulic failure defines the recovery and point of death in water-stressed conifers. Plant Physiol. 149 , 575–584 (2009).
Bassiouni, M., Good, S. P., Still, C. J. & Higgins, C. W. Plant water uptake thresholds inferred from satellite soil moisture. Geophys. Res. Lett. 47 , e2020GL087077 (2020).
Jonard, F., Feldman, A. F., Gianotti, D. J. S. & Entekhabi, D. Observed water- and light-limitation across global ecosystems. Biogeosciences 19 , 5575–5590 (2022).
Short Gianotti, D. J., Rigden, A. J., Salvucci, G. D. & Entekhabi, D. Satellite and station observations demonstrate water availability’s effect on continental-scale evaporative and photosynthetic land surface dynamics. Water Resour. Res. 55 , 540–554 (2019).
Feldman, A. F., Short Gianotti, D. J., Trigo, I. F., Salvucci, G. D. & Entekhabi, D. Satellite-based assessment of land surface energy partitioning–soil moisture relationships and effects of confounding variables. Water Resour. Res. 55 , 10657–10677 (2019).
Schwinning, S. & Sala, O. E. Hierarchy of responses to resource pulses in arid and semi-arid ecosystems. Oecologia 141 , 211–220 (2004).
Famiglietti, C. A., Michalak, A. M. & Konings, A. G. Extreme wet events as important as extreme dry events in controlling spatial patterns of vegetation greenness anomalies. Environ. Res. Lett. 16 , 074014 (2021).
Novick, K. A. et al. Confronting the water potential information gap. Nat. Geosci. 15 , 158–164 (2022).
Feldman, A. F., Short Gianotti, D. J., Konings, A. G., Gentine, P. & Entekhabi, D. Patterns of plant rehydration and growth following pulses of soil moisture availability. Biogeosciences 18 , 831–847 (2021).
Post, A. K. & Knapp, A. K. Plant growth and aboveground production respond differently to late-season deluges in a semi-arid grassland. Oecologia 191 , 673–683 (2019).
Huxman, T. E. et al. Response of net ecosystem gas exchange to a simulated precipitation pulse in a semi-arid grassland: the role of native versus non-native grasses and soil texture. Oecologia 141 , 295–305 (2004).
Raz-Yaseef, N., Yakir, D., Schiller, G. & Cohen, S. Dynamics of evapotranspiration partitioning in a semi-arid forest as affected by temporal rainfall patterns. Agric. For. Meteorol. 157 , 77–85 (2012).
Klein, T., Cohen, S. & Yakir, D. Hydraulic adjustments underlying drought resistance of Pinus halepensis . Tree Physiol. 31 , 637–648 (2011).
Feldman, A. F., Chulakadabba, A., Short Gianotti, D. J. & Entekhabi, D. Landscape-scale plant water content and carbon flux behavior following moisture pulses: from dryland to mesic environments. Water Resour. Res. 57 , e2020WR027592 (2021).
Potts, D. L., Barron-Gafford, G. A. & Scott, R. L. Ecosystem hydrologic and metabolic flashiness are shaped by plant community traits and precipitation. Agric. For. Meteorol. 279 , 107674 (2019).
Guo, Q. et al. Responses of gross primary productivity to different sizes of precipitation events in a temperate grassland ecosystem in Inner Mongolia, China. J. Arid Land 8 , 36–46 (2016).
Williams, C. A., Hanan, N., Scholes, R. J. & Kutsch, W. Complexity in water and carbon dioxide fluxes following rain pulses in an African savanna. Oecologia 161 , 469–480 (2009).
Parton, W., Morgan, J., Smith, D. & Grosso, S. D. E. L. Impact of precipitation dynamics on net ecosystem productivity. Glob. Chang. Biol. 18 , 915–927 (2012).
Scott, R. L., Biederman, J. A., Hamerlynck, E. P. & Barron-Gafford, G. A. The carbon balance pivot point of southwestern U.S. semiarid ecosystems: insights from the 21st century drought. J. Geophys. Res. Biogeosci. 120 , 2612–2624 (2015).
Pastorello, G., Trotta, C. & Canfora, E. The FLUXNET2015 dataset and the ONEFlux processing pipeline for eddy covariance data. Sci. Data 7 , 225 (2020).
Post, A. K. & Knapp, A. K. The importance of extreme rainfall events and their timing in a semi-arid grassland. J. Ecol. 108 , 2431–2443 (2020).
Collins, S. L. et al. A multiscale, hierarchical model of pulse dynamics in arid-land ecosystems. Annu. Rev. Ecol. Evol. Syst. 45 , 397–419 (2014).
Feldman, A. F. et al. Moisture pulse-reserve in the soil-plant continuum observed across biomes. Nat. Plants 4 , 1026–1033 (2018). An evaluation of plant sensitivity to individual rainfall pulses and their soil moisture thresholds using satellite measurements across the tropics.
Harris, B. L. et al. Satellite-observed vegetation responses to intraseasonal precipitation variability. Geophys. Res. Lett. 49 , 1–11 (2022).
Roman, D. T. et al. The role of isohydric and anisohydric species in determining ecosystem-scale response to severe drought. Oecologia 179 , 641–654 (2015).
Ignace, D. D., Huxman, T. E., Weltzin, J. F. & Williams, D. G. Leaf gas exchange and water status responses of a native and non-native grass to precipitation across contrasting soil surfaces in the Sonoran Desert. Oecologia 152 , 401–413 (2007).
Craine, J. M. et al. Timing of climate variability and grassland productivity. Proc. Natl Acad. Sci. USA 109 , 3401–3405 (2012).
Hao, Y. B. et al. Aboveground net primary productivity and carbon balance remain stable under extreme precipitation events in a semiarid steppe ecosystem. Agric. For. Meteorol. 240–241 , 1–9 (2017).
Brown, R. F., Sala, O. E., Sinsabaugh, R. L. & Collins, S. L. Temporal effects of monsoon rainfall pulses on plant available nitrogen in a Chihuahuan desert grassland. J. Geophys. Res. Biogeosci. 127 , e2022JG006938 (2022).
Sitch, S. et al. Evaluation of ecosystem dynamics, plant geography and terrestrial carbon cycling in the LPJ dynamic global vegetation model. Glob. Chang. Biol. 9 , 161–185 (2003).
Zhang, F. et al. Using high frequency digital repeat photography to quantify the sensitivity of a semi-arid grassland ecosystem to the temporal repackaging of precipitation. Agric. For. Meteorol. 338 , 109539 (2023).
Paschalis, A. et al. Rainfall manipulation experiments as simulated by terrestrial biosphere models: where do we stand? Glob. Chang. Biol. 26 , 3336–3355 (2020).
McColl, K. A. et al. The global distribution and dynamics of surface soil moisture. Nat. Geosci. 10 , 100–104 (2017).
Cherwin, K. & Knapp, A. Unexpected patterns of sensitivity to drought in three semi-arid grasslands. Oecologia 169 , 845–852 (2012).
Cochard, H., Bréda, N. & Granier, A. Whole tree hydraulic conductance and water loss regulation in Quercus during drought: evidence for stomatal control of embolism? Ann. For. Sci. 53 , 197–206 (1996).
Medlyn, B. E. et al. Reconciling the optimal and empirical approaches to modelling stomatal conductance. Glob. Chang. Biol. 17 , 2134–2144 (2011).
Berdugo, M. et al. Global ecosystem thresholds driven by aridity. Science 367 , 787–790 (2020).
North, G. B. & Nobel, P. S. Changes in hydraulic conductivity and anatomy caused by drying and rewetting roots of agave deserti (Agavaceae). Am. J. Bot. 78 , 906 (1991).
McDowell, N. G. et al. Mechanisms of woody-plant mortality under rising drought, CO 2 and vapour pressure deficit. Nat. Rev. Earth Environ. 3 , 294–308 (2022).
Choat, B. et al. Global convergence in the vulnerability of forests to drought. Nature 491 , 752–755 (2012).
Gherardi, L. A. & Sala, O. E. Effect of interannual precipitation variability on dryland productivity: a global synthesis. Glob. Chang. Biol. 25 , 269–276 (2019).
Konings, A. G. & Gentine, P. Global variations in ecosystem-scale isohydricity. Glob. Chang. Biol. 23 , 891–905 (2017).
Still, C. J., Berry, J. A., Collatz, G. J. & DeFries, R. S. Global distribution of C3 and C4 vegetation: carbon cycle implications. Global Biogeochem. Cycles 17 , 1006 (2003).
Yu, K. & D’Odorico, P. Climate, vegetation, and soil controls on hydraulic redistribution in shallow tree roots. Adv. Water Resour. 66 , 70–80 (2014).
Larsen, L., Thomas, C., Eppinga, M. & Coulthard, T. Exploratory modeling: extracting causality from complexity. Eos Trans. Am. Geophys. Union 95 , 285–286 (2014).
Weltzin, J. F. et al. Assessing the response of terrestrial ecosystems to potential changes in precipitation. Bioscience 53 , 941 (2003).
Kröel-Dulay, G. et al. Field experiments underestimate aboveground biomass response to drought. Nat. Ecol. Evol. 6 , 540–545 (2022).
Novick, K. A. et al. The increasing importance of atmospheric demand for ecosystem water and carbon fluxes. Nat. Clim. Chang. 6 , 1023–1027 (2016).
Lundholm, J. T. & Larson, D. W. Experimental separation of resource quantity from temporal variability: seedling responses to water pulses. Oecologia 141 , 346–352 (2004).
Ogle, K. & Reynolds, J. F. Plant responses to precipitation in desert ecosystems: integrating functional types, pulses, thresholds, and delays. Oecologia 141 , 282–294 (2004).
Orth, R. When the land surface shifts gears. AGU Adv. 2 , 2019–2022 (2021).
Hsu, J. S., Powell, J. & Adler, P. B. Sensitivity of mean annual primary production to precipitation. Glob. Chang. Biol. 18 , 2246–2255 (2012).
Maurer, G. E., Hallmark, A. J., Brown, R. F., Sala, O. E. & Collins, S. L. Sensitivity of primary production to precipitation across the United States. Ecol. Lett. 23 , 527–536 (2020).
Feldman, A. F., Gianotti, D. J. S., Trigo, I. F., Salvucci, G. D. & Entekhabi, D. Observed landscape responsiveness to climate forcing. Water Resour. Res. 58 , e2021WR030316 (2022).
Dong, J., Akbar, R., Feldman, A., Gianotti, D. S. & Entekhabi, D. Land surfaces at the tipping-point for water and energy balance coupling. Water Resour. Res. 59 , e2022WR032472 (2023).
Fu, Z. et al. Critical soil moisture thresholds of plant water stress in terrestrial ecosystems. Sci. Adv. 7827 , 1–13 (2022).
Fu, Z. et al. Uncovering the critical soil moisture thresholds of plant water stress for European ecosystems. Glob. Chang. Biol. 28 , 2111–2123 (2022).
Jensen, J. On the convex functions and inequalities between mean values. Acta Math. 30 , 175–193 (1906).
Heisler-White, J. L., Knapp, A. K. & Kelly, E. F. Increasing precipitation event size increases aboveground net primary productivity in a semi-arid grassland. Oecologia 158 , 129–140 (2008).
Guan, K., Sultan, B., Biasutti, M., Baron, C. & Lobell, D. B. What aspects of future rainfall changes matter for crop yields in West Africa? Geophys. Res. Lett. 42 , 8001–8010 (2015).
Seyfried, M. S. et al. Ecohydrological control of deep drainage in arid and semiarid regions. Ecology 86 , 277–287 (2005).
Sala, O. E., Gherardi, L. A. & Peters, D. P. C. Enhanced precipitation variability effects on water losses and ecosystem functioning: differential response of arid and mesic regions. Clim. Change 131 , 213–227 (2015).
Berkelhammer, M., Stefanescu, I. C., Joiner, J. & Anderson, L. High sensitivity of gross primary production in the Rocky mountains to summer rain. Geophys. Res. Lett. 44 , 3643–3652 (2017).
Nielsen, U. N. & Ball, B. A. Impacts of altered precipitation regimes on soil communities and biogeochemistry in arid and semi-arid ecosystems. Glob. Chang. Biol. 21 , 1407–1421 (2015).
Biederman, J. A. et al. Terrestrial carbon balance in a drier world: the effects of water availability in southwestern North America. Glob. Chang. Biol. 22 , 1867–1879 (2016).
Siteur, K. et al. How will increases in rainfall intensity affect semiarid ecosystems? Water Resour. Res. 50 , 5980–6001 (2014).
Li, L. et al. Nonlinear carbon cycling responses to precipitation variability in a semiarid grassland. Sci. Total Environ. 781 , 147062 (2021).
Swemmer, A. M., Knapp, A. K. & Snyman, H. A. Intra-seasonal precipitation patterns and above-ground productivity in three perennial grasslands. J. Ecol. 95 , 780–788 (2007).
Fu, Z. et al. Atmospheric dryness reduces photosynthesis along a large range of soil water deficit. Nat. Commun. 13 , 989–999 (2022).
Rigden, A. J., Mueller, N. D., Holbrook, N. M., Pillai, N. & Huybers, P. Combined influence of soil moisture and atmospheric evaporative demand is important for accurately predicting US maize yields. Nat. Food 1 , 127–133 (2020).
Kannenberg, S. A. et al. Quantifying the drivers of ecosystem fluxes and water potential across the soil–plant–atmosphere continuum in an arid woodland. Agric. For. Meteorol. 329 , 109269 (2023).
Konings, A. G., Williams, A. P. & Gentine, P. Sensitivity of grassland productivity to aridity controlled by stomatal and xylem regulation. Nat. Geosci. 10 , 284–288 (2017).
Zscheischler, J. et al. A few extreme events dominate global interannual variability in gross primary production. Environ. Res. Lett. 9 , 035001 (2014).
Zscheischler, J. et al. Short-term favorable weather conditions are an important control of interannual variability in carbon and water fluxes. J. Geophys. Res. Biogeosci. 121 , 2186–2198 (2016).
Smith, M. D. The ecological role of climate extremes: current understanding and future prospects. J. Ecol. 99 , 651–655 (2011).
Smith, M. D. An ecological perspective on extreme climatic events: a synthetic definition and framework to guide future research. J. Ecol. 99 , 656–663 (2011).
Knapp, A. K. et al. Characterizing differences in precipitation regimes of extreme wet and dry years: implications for climate change experiments. Glob. Chang. Biol. 21 , 2624–2633 (2015).
Sala, O. E. & Lauenroth, W. K. Small rainfall events: an ecological role in semiarid regions. Oecologia 53 , 301–304 (1982).
Rezaei, E. E. et al. Climate change impacts on crop yields. Nat. Rev. Earth Environ. 4 , 831–846 (2023).
Donohue, R. J., McVicar, T. R. & Roderick, M. L. Climate-related trends in Australian vegetation cover as inferred from satellite observations, 1981–2006. Glob. Chang. Biol. 15 , 1025–1039 (2009).
Haverd, V., Ahlström, A., Smith, B. & Canadell, J. G. Carbon cycle responses of semi-arid ecosystems to positive asymmetry in rainfall. Glob. Chang. Biol. 23 , 793–800 (2017).
Copernicus Climate Change Service Climate Data Store. CMIP6 climate projections. C3S https://doi.org/10.24381/cds.c866074c (2021).
Eyring, V. et al. Overview of the Coupled Model Intercomparison Project Phase 6 (CMIP6) experimental design and organization. Geosci. Model Dev. 9 , 1937–1958 (2016).
Fatichi, S., Ivanov, V. Y. & Caporali, E. Investigating interannual variability of precipitation at the global scale: is there a connection with seasonality? J. Clim. 25 , 5512–5523 (2012).
Hajek, O. L. & Knapp, A. K. Shifting seasonal patterns of water availability: ecosystem responses to an unappreciated dimension of climate change. N. Phytol. 233 , 119–125 (2022).
Paschalis, A., Fatichi, S., Katul, G. G. & Ivanov, V. Y. Cross-scale impact of climate temporal variability on ecosystem water and carbon fluxes. J. Geophys. Res. Biogeosci. 120 , 641–660 (2015).
Guo, Q. et al. Spatial variations in aboveground net primary productivity along a climate gradient in Eurasian temperate grassland: effects of mean annual precipitation and its seasonal distribution. Glob. Chang. Biol. 18 , 3624–3631 (2012).
Chou, C. et al. Increase in the range between wet and dry season precipitation. Nat. Geosci. 6 , 263–267 (2013).
Good, S. P., Guan, K. & Caylor, K. K. Global patterns of the contributions of storm frequency, intensity, and seasonality to interannual variability of precipitation. J. Clim. 29 , 3–15 (2016).
Download references
Acknowledgements
The research of A.F.F. was supported by an appointment to the NASA Postdoctoral Program at the NASA Goddard Space Flight Center, administered by Oak Ridge Associated Universities under contract with NASA. A.F.F. was partly supported by a NASA Terrestrial Ecology scoping study for a dryland field campaign. A.G.K. was supported by NSF DEB 1942133 and by the Alfred P. Sloan Foundation. The authors acknowledge the World Climate Research Programme, which, through its Working Group on Coupled Modelling, coordinated and promoted CMIP6. The authors thank the climate modelling groups for producing and making available their model output, the Earth System Grid Federation (ESGF) for archiving the data and providing access and the multiple funding agencies who support CMIP6 and ESGF. This work used eddy covariance data acquired and shared by the FLUXNET community, including AmeriFlux. The FLUXNET eddy covariance data processing and harmonization was carried out by the ICOS Ecosystem Thematic Center, AmeriFlux Management Project and Fluxdata project of FLUXNET, with the support of CDIAC, and the OzFlux, ChinaFlux and AsiaFlux offices.
Author information
Authors and affiliations.
Biospheric Sciences Laboratory, NASA Goddard Space Flight Center, Greenbelt, MD, USA
Andrew F. Feldman & Benjamin Poulter
Earth System Science Interdisciplinary Center, University of Maryland, College Park, MD, USA
Andrew F. Feldman
Department of Civil, Environmental and Geo-Engineering, University of Minnesota, Minneapolis, MN, USA
Saint Anthony Falls Laboratory, University of Minnesota, Minneapolis, MN, USA
Department of Land Resources and Environmental Sciences, Montana State University, Bozeman, MT, USA
Andrew J. Felton
Department of Earth System Science, Stanford University, Stanford, CA, USA
Alexandra G. Konings
Department of Biology and Graduate Degree Program in Ecology, Colorado State University, Fort Collins, CO, USA
Alan K. Knapp
USDA Agricultural Research Service Southwest Watershed Research Center, Tucson, AZ, USA
Joel A. Biederman
You can also search for this author in PubMed Google Scholar
Contributions
A.F.F. led the Review and wrote the initial draft. X.F., A.J.F., A.G.K, A.K.K, J.A.B. and B.P. all contributed substantial edits to the initial outline, manuscript and figures. All authors contributed equally to the generation of the central ideas in the manuscript and initial figure concepts.
Corresponding author
Correspondence to Andrew F. Feldman .
Ethics declarations
Competing interests.
The authors declare no competing interests.
Peer review
Peer review information.
Nature Reviews Earth & Environment thanks Madelon Case, Rene Orth, Yuting Yang and Yao Zhang for their contribution to the peer review of this work.
Additional information
Publisher’s note Springer Nature remains neutral with regard to jurisdictional claims in published maps and institutional affiliations.
Supplementary information
Supplementary information.
An evolutionary adaptation of photosynthesis occurring mainly in some grass and crop species under which photosynthesis is more efficient because photorespiration is largely avoided.
An evolutionary adaptation of photosynthesis occurring mainly in plant species in arid environments that allows them to save water by only exchanging gases with the atmosphere at night.
The species types and their relative abundance within a defined ecosystem, here referring specifically to plants.
A thermodynamic equation that describes the nonlinear increase of saturation vapour pressure, or the capacity of air to hold water, with increases in air temperature.
Some of the largest organized circulations of air in the atmosphere of the Earth that contribute substantially to weather and climate patterns of the Earth.
Rainfall that is captured and stored by vegetation, even briefly, such that it is prevented from infiltrating into the soil or running off of the ground surface.
A commonly used satellite-based vegetation index that estimates greenness at the top of the vegetation canopy based on satellite measurements in the infrared portion of the electromagnetic spectrum.
The annual cyclic nature of plant functioning, specifically referring to its periodic increase and decrease in functioning during similar months of each year.
A general indicator based on how much water is available for plants to use towards essential plant functions such as photosynthesis and transpiration.
The rainfall rate or rainfall depth over a defined time period. The rainfall rate is often defined hourly across hydrological sciences, although it is designated to be daily in this Review.
The distribution of the root volume of a plant across the soil depth.
Vegetation metrics derived from satellite measurements that typically span large spatial extents, including vegetation areal cover, greenness, height, photosynthetic capacity, water content and others.
An empirical relationship between decreasing soil moisture and decline in plant functions such as photosynthesis or transpiration.
Rights and permissions
Springer Nature or its licensor (e.g. a society or other partner) holds exclusive rights to this article under a publishing agreement with the author(s) or other rightsholder(s); author self-archiving of the accepted manuscript version of this article is solely governed by the terms of such publishing agreement and applicable law.
Reprints and permissions
About this article
Cite this article.
Feldman, A.F., Feng, X., Felton, A.J. et al. Plant responses to changing rainfall frequency and intensity. Nat Rev Earth Environ 5 , 276–294 (2024). https://doi.org/10.1038/s43017-024-00534-0
Download citation
Accepted : 23 February 2024
Published : 09 April 2024
Issue Date : April 2024
DOI : https://doi.org/10.1038/s43017-024-00534-0
Share this article
Anyone you share the following link with will be able to read this content:
Sorry, a shareable link is not currently available for this article.
Provided by the Springer Nature SharedIt content-sharing initiative
Quick links
- Explore articles by subject
- Guide to authors
- Editorial policies
Sign up for the Nature Briefing newsletter — what matters in science, free to your inbox daily.

Find Info For
- Current Students
- Prospective Students
- Research and Partnerships
- Entrepreneurship and Commercialization
Quick Links
- Health and Life Sciences
- Info Security and AI
- Transformative Education
- Purdue Today
- Purdue Global
- Purdue in the News
April 10, 2024
‘Citi-Sci: Gardening for Science!’ experiment focuses on gardening preferences and effects on mental health, seeks participants
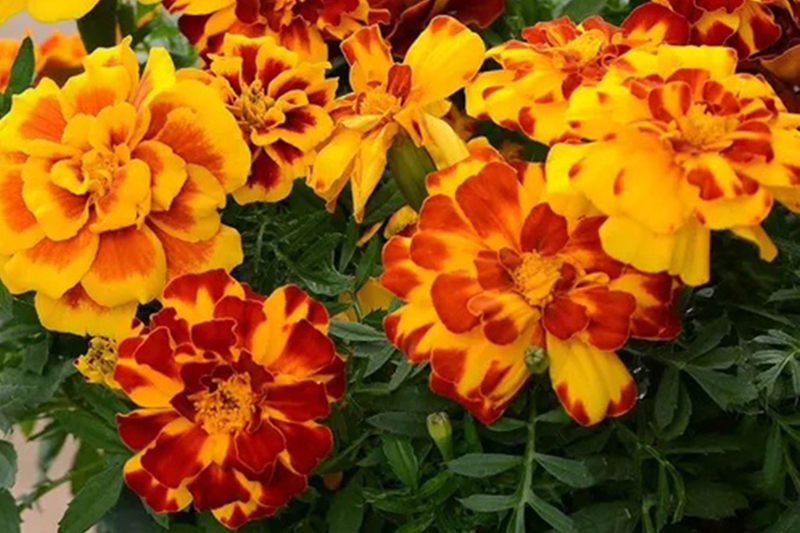
Gardeners at any level are invited to join Purdue University Extension, the University of Tennessee, Iowa State University and North Carolina State University from April through September for the third year of the citizen-science experiment called “Citi-Sci: Gardening for Science!” (Photo provided by Michael Fidler)
WEST LAFAYETTE, Ind. — Gardeners at any level are invited to join Purdue University Extension , the University of Tennessee, Iowa State University and North Carolina State University for the third year of the citizen-science experiment called “Citi-Sci: Gardening for Science!” from April through September 2024.
Gardeners or citizen-scientists who participate, dubbed “citi-scientists,” will have the opportunity to grow four different types of plants — two strawberry and two marigold plants — some with edible flowers.
Celina Gomez , associate professor of controlled environment agriculture in Purdue’s Department of Horticulture and Landscape Architecture and experiment coordinator, seeks to gain information for their industry stakeholders, who are greenhouse growers. She says, “We want to better understand consumer preferences and challenges with gardening. For example, is there value in selling containers of strawberry or marigold plants with different colors versus one color?”
Data will be collected through surveys. Plants will be provided at no cost to citi-scientists helping to gauge gardener preferences and challenges. Along with the plants and other materials needed for the experiment, participants will have access to monthly educational sessions led by researchers from each institution’s extension department. Session topics will cover pest management, healthy cooking and nutrition, and mental health.
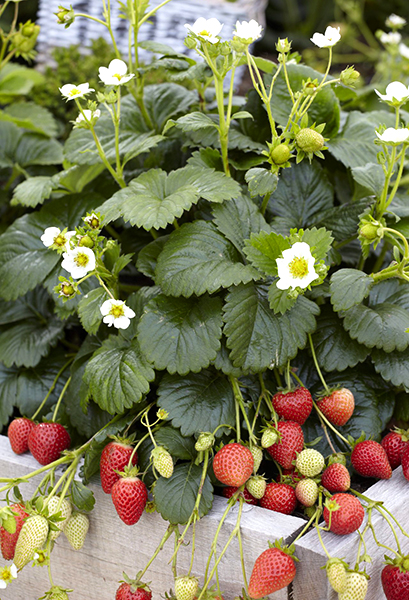
“This experiment is a chance for the community to be involved in science,” says Michael Fidler , Purdue horticulture and landscape architecture master’s student and graduate research assistant, who leads the experiment with Gomez. “While the data we collect and research we conduct in this field is valuable, we sometimes forget about the human aspect. This is a chance to take part in the scientific process.”
Gomez adds, “This year, we will also focus more on the psychological aspects of gardening, specifically how participating in a community-oriented project like this can positively impact your mental health.”
Those interested in participating can sign up online by April 26. The experiment is open to all, requiring only a small, sunny place for plants to grow from each citi-scientist. If selected for the research project, participants will receive plant pickup dates, location details and more information with additional resources. Contact Gomez at [email protected] or 765-494-4443 for any questions.
About Purdue University
Purdue University is a public research institution demonstrating excellence at scale. Ranked among top 10 public universities and with two colleges in the top four in the United States, Purdue discovers and disseminates knowledge with a quality and at a scale second to none. More than 105,000 students study at Purdue across modalities and locations, including nearly 50,000 in person on the West Lafayette campus. Committed to affordability and accessibility, Purdue’s main campus has frozen tuition 13 years in a row. See how Purdue never stops in the persistent pursuit of the next giant leap — including its first comprehensive urban campus in Indianapolis, the new Mitchell E. Daniels, Jr. School of Business, and Purdue Computes — at https://www.purdue.edu/president/strategic-initiatives .
Writer/Media contact: Ashvini Malshe, 765-496-7480, [email protected]
Source: Celina Gomez, 765-494-4443, [email protected]
Agricultural Communications: 765-494-8415;
Maureen Manier, Department Head, [email protected]
Agriculture News Page
Communication
- OneCampus Portal
- Brightspace
- BoilerConnect
- Faculty and Staff
- Human Resources
- Colleges and Schools
Info for Staff
- Purdue Moves
- Board of Trustees
- University Senate
- Center for Healthy Living
- Information Technology
- Ethics & Compliance
- Campus Disruptions
Purdue University, 610 Purdue Mall, West Lafayette, IN 47907, (765) 494-4600
© 2015-24 Purdue University | An equal access/equal opportunity university | Copyright Complaints | Maintained by Office of Strategic Communications
Trouble with this page? Disability-related accessibility issue? Please contact News Service at [email protected] .

Study shedding new light on Earth's global carbon cycle could help assess liveability of other planets
Research has uncovered important new insights into the evolution of oxygen, carbon, and other vital elements over the entire history of Earth – and it could help assess which other planets can develop life, ranging from plants to animals and humans.
The study, published today in Nature Geoscience and led by a researcher at the University of Bristol, reveals for the first time how the build up of carbon-rich rocks has accelerated oxygen production and its release into the atmosphere. Until now the exact nature of how the atmosphere became oxygen-rich has long eluded scientists and generated conflicting explanations.
As carbon dioxide is steadily emitted by volcanoes, it ends up entering the ocean and forming rocks like limestone. As global stocks of these rocks build up they can then release their carbon during tectonic processes, including mountain building and metamorphism.
Using this knowledge, the scientists built a unique sophisticated computer model to more accurately chart key changes in the carbon, nutrient and oxygen cycles deep into Earth’s history, over 4 billion years of the planet’s lifetime.
Lead author and biogeochemist Dr Lewis Alcott, Lecturer in Earth Sciences at the University of Bristol, said: “This breakthrough is important and exciting because it may help us understand how planets, other than Earth, have the potential to support intelligent, oxygen-breathing life.
“Previously we didn’t have a clear idea of why oxygen rose from very low concentrations to present-day concentrations, as computer models haven’t previously been able to accurately simulate all the possible feedbacks together. This has puzzled scientists for decades and created different theories.”
The discovery indicates that older planets, originating billions of years ago like Earth, may have better prospects to accumulate enough carbon-rich deposits in their crust, which could facilitate rapid recycling of carbon and nutrients for life.
The findings showed this gradual carbon enrichment of the crust results in ever-increasing recycling rates of carbon and various minerals, including the nutrients needed for photosynthesis, the process green plants use sunlight to absorb nutrients from carbon dioxide and water. This cycle therefore steadily speeds up oxygen production over the passage of Earth’s history.
The research, which started whilst Dr Alcott was a Hutchinson Postdoctoral Fellow at Yale University in the United States, paves the way for future work to further unravel the complex interrelationships between planetary temperature, oxygen, and nutrients.
Co-author Prof Benjamin Mills, Professor of Earth System Evolution at the University of Leeds, said: “We have lots of information about distant stars and the size of the planets that orbit them. Soon this could be used to make a prediction of the planet’s potential chemistry, and new advances in telescope technology should let us know if we are correct."
- Extrasolar Planets
- Astrophysics
- Asteroids, Comets and Meteors
- Kuiper Belt
- Planetary habitability
- Planetary nebula
- Extraterrestrial life
- Near-Earth asteroid
- Gravitation
- History of Earth
- Extrasolar planet
Story Source:
Materials provided by University of Bristol . Note: Content may be edited for style and length.
Journal Reference :
- Lewis J. Alcott, Craig Walton, Noah J. Planavsky, Oliver Shorttle, Benjamin J. W. Mills. Crustal carbonate build-up as a driver for Earth’s oxygenation . Nature Geoscience , 2024; DOI: 10.1038/s41561-024-01417-1
Cite This Page :
Explore More
- Pacific Cities Much Older Than Previously ...
- The Milky Way in Ancient Egyptian Mythology
- Physical Activity Best in the Evening
- How the Body Switches out of 'Fight' Mode
- New Drug Prevents Flu-Related Lung Damage
- 3D Mouth of an Ancient Jawless Fish
- Connecting Lab-Grown Brain Cells
- Device: Self-Healing Materials, Drug Delivery
- How We Perceive Bitter Taste
- Next-Generation Digital Displays
Trending Topics
Strange & offbeat.

O.J. Simpson died after his battle with cancer, according to his family.
- LIFE AFTER ACQUITTAL
25 years later: Nature still healing from Tri-State tornado that killed 4
by Chelsea Sick, WKRC

MONTGOMERY, Ohio (WKRC) - It's been 25 years since an EF-4 tornado ripped through Blue Ash and Montgomery.
Four people died that day in 1999. The storm had winds in excess of 200 mph., demolishing at least 200 homes and damaging twice that. Despite the devastation of that day in 1999, you may not notice anything out of place when you drive through the area today, that's unless you go on a hike.
"In some areas, it just looked liked somebody took their hand and swiped all the houses down," said Retired Deputy Fire Chief Terry Ott.
Ott was one of the first on scene after the storm hit. He was working for the Deer Park Silverton Joint Fire District. He helped with search and rescue on Cornell Road.
"It was just unbelievable, and you know the initial shock of seeing that. It's like, where do you start?" Ott said.
Homes on Cornell Road have been rebuilt and families have recovered, but 25 years later, nature is still healing.
"We always just assume nature is just going to rebound and resurge and be okay," said Dr. Theresa Culley.
Dr. Culley is a professor of plant biology at the University of Cincinnati. She led UC's long-term study on the UC Harris Benedict Nature Preserve that was damaged in that storm.
Those studies found forests can regenerate after huge storms, but they tend to have fewer native species and more invasive species.
"At the top of that tree, that's a bad plant. That's an invasive [plant] that's called garlic mustard," Dr. Culley said.
Volunteer and student groups periodically visit the preserve to remove invasive species, like honeysuckle. Dr. Culley said that the fewer invasive species, the more native plants, which keep animals in the habitat healthier. She said you find more invasive species in urban preserves because people have invasive ground cover in their gardens.
"When it stays in the yard, it's a great plant. But when it gets into natural areas and it forms these mats and so, that could be a problem," Dr. Culley said.
In cases like this, it's slowing down recovery.
"We have to do a better job of educating," Dr. Culley said.
While the people have rebuilt, Dr. Culley said nature's recovery could be studied for decades to come.

An official website of the United States government
The .gov means it’s official. Federal government websites often end in .gov or .mil. Before sharing sensitive information, make sure you’re on a federal government site.
The site is secure. The https:// ensures that you are connecting to the official website and that any information you provide is encrypted and transmitted securely.
- Publications
- Account settings
Preview improvements coming to the PMC website in October 2024. Learn More or Try it out now .
- Advanced Search
- Journal List
- Plants (Basel)

Is There a Role for Sound in Plants?
Associated data.
Not applicable.
Plants have long been considered passive, static, and unchanging organisms, but this view is finally changing. More and more knowledge is showing that plants are aware of their surroundings, and they respond to a surprising variety of stimuli by modifying their growth and development. Plants extensively communicate with the world around them, above and below ground. Although communication through mycorrhizal networks and Volatile Organic Compounds has been known for a long time, acoustic perception and communication are somehow a final frontier of research. Perhaps surprisingly, plants not only respond to sound, they actually seem to emit sound as well. Roots emit audible clicks during growth, and sounds are emitted from xylem vessels, although the nature of these acoustic emissions still needs to be clarified. Even more interesting, there is the possibility that these sounds carry information with ecological implications, such as alerting insects of the hydration state of a possible host plant, and technological implications as well. Monitoring sound emissions could possibly allow careful monitoring of the hydration state of crops, which could mean significantly less water used during irrigation. This review summarizes the current knowledge on sound perception communication in plants and illustrates possible implications and technological applications.
1. Introduction
Plants have been around for a long time, far longer than Homo sapiens . Homo sapiens arose approximately 300,000 years ago [ 1 ], a minimal length of time compared with the age of Earth. The earliest land plants, on the other hand, first appeared in the fossil record millions of years prior to this. Non-vascular plants such as the true mosses, the Bryopsida, for example, first appeared in the Mississippian 340 million years ago and appeared well established by the Permian. However, the earliest bryophytes in the fossil record already have the basic thallus organization possessed by current forms, suggesting the possibility that the bryophytes evolved even earlier than the fossil record suggests [ 2 ]. Baragwanathia, which is a relatively complex vascular plant, was confirmed to be of Late Silurian origin, which was approximately 420 million years ago [ 2 ]. Fossils of the first flowering plants date back to around 130 million years ago, a milestone not only for their role in the evolution of plants but also for the relationship of plants with insects [ 3 ]. The appearance, enormous diversification, and ecological radiation of the angiosperms began during the Cretaceous, between 135 to 65 million years ago, and it represented a very significant alteration to the history of life on Earth. It had vast repercussions on the distribution of other groups of land plants and a great effect on the evolution of ecosystems and species. Today, there are more than 350,000 species of extant angiosperms, which is more than all the other groups of land plants combined [ 4 ]. They occupy and dominate an astounding range of habitats, and -as autotrophs- the angiosperms are the base upon which most ecosystems are built [ 4 ].
Plants are sessile and photoautotrophic, which means that they produce new biomass from CO 2 using light energy in a process called photosynthesis. Most of the energy that enters terrestrial habitats is the result of photosynthesis. Plants are so important that they go so far as to influence the atmosphere and climate, and yet at the same time, the environment itself has a profound impact on photosynthesis and plants.
And therein lies the point. Plants are not passive organisms, though their sessile nature might make them appear to be static or unchanging. Though plants have an awe-inspiring impact on most life on earth, most people tend to underappreciate or not notice the plants living around them. Plants are alive, and like all things that are alive, they perceive many environmental and physiological signals, and through these, they perfect and modify their growth and development. Not only that, but recent scientific studies have also shown that plants are capable of exhibiting learning, memory, and even intelligence (although maybe not consciousness) [ 5 , 6 , 7 , 8 ]. It is reasonable to assume that plants have evolved to do so. After all, as stated before, land plants first appeared millions of years ago. As new plant forms evolved, so did the capacity to perceive stimuli and adapt to a changing environment [ 9 ].
But plants do much more than just perceive and react. Plants communicate amongst themselves and with animals. Plants do so in many ways, both above and below ground; a growing body of research shows that plants can even detect and emit sounds (for a review, see [ 10 , 11 ]).
2. Communication through Sound
A growing body of research is showing that plants detect and emit sounds. This is unsurprising considering that there is no habitat colonized by plants that is without sound and taking into account their sessile nature and their age on Earth, it is reasonable to think that they have learned to do so.
2.1. What Is “Sound”
Briefly, sound is defined as a series of longitudinal waves of pressure that propagate through compressible media, such as air, liquids, or solids. Sound waves that fall in the range of frequencies between 20 Hz and 20 kHz belong to the audible sound, which is what a human ear can hear; frequencies below 20 Hz or higher than 20 kHz are defined as infrasound or ultrasound, respectively [ 12 ]. However, sound is not only vibration energy; it is also pressure generated by vibration waves that move through a suitable medium in the form of compression and rarefaction [ 12 ]. For a sound to be perceived, however, it is not sufficient that it consists of frequencies in the audible range; it must also have sufficient sound pressure, that is, the pressure variation produced by the acoustic phenomenon compared with the static value. For example, in the air a sound pressure of 20 µPa corresponds to a level of sound pressure of 0 dB [ 13 ].
Application of sound at different frequencies, pressure levels, duration, and repetition of exposure periods has been proved to have an influence on plant growth, development, and germination [ 10 ]. Taken together, these studies belong to the field of Plant Acoustics. Whether or not sound perception and/or emission are used in plant communication is a fascinating field of research. However, for acoustic plant communication to exist, there needs to be an emitter, in other words, a source of sound and a receiver not only able to perceive the signal but also able to decipher and eventually perform a sort of coherent response.
Sound stimulation has been proven to switch on stress-induced genes [ 14 ] or enhance genes related to disease resistance [ 15 ], but there is also a sort of new age interest in growing plants with sound. Traditional ethnic music can positively affect the productivity and quality of plants. Many studies are heading in that direction: Javanese music has been applied to Chinese broccoli ( Brassica alboglabra ) plants [ 16 ], and Desmodyium girans (Telegraph plant) [ 17 ] and rice ( Oryza sativa ) [ 18 ] show better growth performance when exposed to Buddhist pirith chants.
2.2. Sound at Cellular and Subcellular Level
On a cellular level, it seems that the most likely candidate for sound signaling is Ca 2+ , which acts as a second messenger to Sound Vibrations (SVs). Although direct evidence is lacking, it is possible that SVs activate plasma membrane channels, evoking a membrane potential-based signaling cascade. Several studies have shown an efflux/influx of Ca 2+ following SVs. It was shown, for example, that Chrysanthemum cells treated for one hour with SVs of 100 dB and 1000 Hz had an increase in H + -ATPase activity [ 19 ]. The upstream component of this increased activity was found to be Ca 2+ . It appears that transient increases in cytosolic Ca 2+ concentrations lead to an activation of calcium-dependent protein kinases, which then activate the H + -ATPases. These kinases go on to regulate proteins and transcription factors, therefore altering gene expression [ 20 , 21 , 22 , 23 ]. For example, after treatment with SVs, plant cells showed increases in α-amylase activity and as a result, an increase in sugar levels. ROS scavenging enzymes have been shown to increase activity after SV treatment, and overall, the most common plant response to SV treatment is increased growth, for example, through increased cell division. All this, however, is just a general overview of what happens at the cellular level following sound perception, and it is well described in greater detail by Mishra and co-workers in 2016 [ 23 ].
But there is another side to the coin. Sound is generated by vibrating objects, and the components of eukaryotic cells do just that. Following the hydrolysis of Adenosine triphosphate (ATP), motor proteins such as myosin generate vibrations [ 24 ]. In addition, the nanomechanical motions of the cell wall of Saccharomyces cerevisiae , baker’s yeast, are in the range of 800–1600 Hz, with amplitudes of 3 nm. Interestingly, exposing the cells of S. cerevisiae to a metabolic inhibitor caused the periodic motion to cease [ 25 ]. Cells are surrounded by other cells so that a cell can be influenced by the mechanical properties of its adjacent cells, and this can build up to a collective mode which results in amplification of the signal [ 24 ].
2.3. Sound like Touch
Sound and touch have similar physical properties, and yet plants cannot only properly distinguish between sound and touch, but they are also able to distinguish between relevant and irrelevant sound. The ability of plants to perceive touch has been well known for a long time. One need only to look at the carnivorous plant Dionaea muscipula or at Mimosa pudica to see plants reacting to touch. Since sound is generated by a vibrating body, and it propagates longitudinally by vibrating the particles of the medium, it passes through, when it reaches a body, it vibrates the body mechanically as well. In other words, sound waves mechanically impact an object they meet on their path [ 9 ].
The molecular basis for the perception of a mechanical stimulus in plants remains to be identified. However, touch sensitivity is not just limited to sensitive plant and carnivorous species. Every plant (or plant cell) perceives and accordingly responds to mechanostimulation [ 26 ]. Even plant roots are extremely sensitive to touch, being able to turn around an obstacle in their path. Like wind, light, rain, touch, sound is a pressure wave that translates into a mechanical influence. For the perception of a mechanical stimulus in plants, Telewski suggested a “unified hypothesis of mechanoperception in plants” [ 27 ] with two models of mechano-receptors: (a) a cytoskeleton based on plasmodesma—plasma membrane—cellular network and (b) ion channels activated by stretching. Given the similarity of the sound stimulus to that of touch, it has recently been seen that signals and perception mechanisms of these two stimuli are common. However, the plants seem to distinguish between the two well in an extraordinarily sophisticated way [ 26 ].
3. Effects of Sound Perception
Human conversation typically has an intensity of approximately 60 dB, and at this intensity, it can elicit vibrations, for example, in hearing organs, of just 10–50 nm. At these scales, the mechanical energy imparted by the vibrations is exceedingly small, and yet we have no problems hearing during conversation. Considering this, it really is reasonable to think that something as small as a trichome could vibrate in response to SVs and possibly convey information [ 28 ].
In much the same way plants have adapted to different pollinators, plants have adapted to different sounds in their environments. For example, flower morphology affects the efficiency of pollinators, affects the way pollinators visit flowers and the success of pollen import and export [ 29 ]. Similarly, the carnivorous pitcher plant Nepenthes hemsleyana could possibly have evolved pitchers that reflect the echolocation of bats. The plant N. hemsleyana has a mutualistic interaction with bats, supplying a safe, parasite-free roosting spot, and the bats in return fertilize the plant with nitrogen-rich droppings, enhancing the nitrogen uptake of these plants by an average of 34% [ 30 ].
3.1. Buzz Pollination
Insects, primarily Hymenoptera [ 31 ], use vibrations to extract pollen from a wide variety of flower morphologies with poricidal anthers, that is, anthers where the pollen exits the anther through an apical pore or slit. This phenomenon is known as buzz pollination. In poricidal anthers, the pollen is not freely accessible, and its removal requires vibration. As many as 8–10% of angiosperms possess poricidal anthers that are pollinated through the use of vibrations. Interestingly, buzz pollination seems to have arisen independently several times in about 65 plant families [ 32 ]. Buzz pollination is not limited to a specific flower morphology, although it seems that the Solanum type flower has evolved specifically in response to sonicating bees. Flowers with poricidal anthers are visited by many insects, even non-sonicating insects that chew through the anthers to reach the pollen, but the primary visitors are sonicating bees [ 31 ].
Sonication seems to have arisen in a common ancestor of bees during the early Cretaceous [ 32 ]. A bee lands on a flower and curls with the ventral side of the body around the anthers in a C shape, with the wings tightly folded back over the abdomen during sonication [ 31 , 33 ]. The bee then rapidly contracts the thoracic muscles while preventing the wings from beating. The vibrations are transmitted to the anthers, which resonate, transmitting energy to the pollen, which is then expelled through the apical aperture [ 31 ]. Centrifugal forces are generated, which eject the pollen [ 34 ].
There are both insect-related and plant-related variables that affect buzz pollination. Vibrations produced by sonicating bees can be characterized by duration, amplitude, and frequency. It was found that the greatest effect on pollen removal from anthers was given by duration and amplitude, while frequency had only a weak effect on pollen removal. Moreover, heavier bees produced buzzes with greater amplitude, ejecting more pollen [ 35 ]. The magnitude of the vibration required to eject pollen from the anthers increased with frequency. The vibration frequency determines the time that a force may act on a particle, and therefore higher frequencies require higher amplitudes [ 34 ].
In terms of duration, bees increased the duration of their buzzing when visiting virgin flowers, and buzzes were shorter when returning to flowers that had already been visited. This could suggest that bees adjust the duration of their buzzing in relation to the pollen content of the flower [ 31 ]. In theory, if a bee vibrated for a long enough time, it could extract all of the available pollen [ 34 ].
The frequency of the buzzing is under physical and physiological control rather than behavioral control. This is because the vibrations depend on the muscles of the bee, and therefore there are limits to the frequencies they can achieve. The peak frequency, which refers to the frequency with the greatest relative energy within a buzzing vibration, varies between 100–400 Hz depending on the species of bee. Through harmonic frequencies, which are positive integer multiples of the original peak frequency (sound-standing waves), frequencies as high as 2000 Hz can be reached [ 31 ], but as stated before, this has very little effect on pollen removal. The optimal peak frequencies do, however, vary among plant species but still remain under 1000 Hz.
Plant traits also affect buzz pollination. Plant structures can either enhance or dampen the amplitude of the vibrations. For example, rigid, multi-layered anthers release more pollen compared with flexible anthers when vibrated. It’s reasonable to think that the size of the apical pore influences the amount of pollen released [ 31 ].
3.2. Sweetened Nectar
Yet another example in the realm of pollination is the production of sweeter nectar within as little as three minutes following the perception of sound by flowers of Oenothera drummondii . The flowers of O. drummondii mechanically vibrated in response to recordings of bees and moths flying and also vibrated in response to the flight of a live bee, showing the same increase in nectar sugar content [ 36 ]. The volume of nectar remained the same, meaning that an increase in sugar concentration was not a result of a drop in water content. The velocities of the oscillations of the flowers that in this experiment caused an increase in sugar concentration in the nectar was found in other experiments to be able to elicit defense responses by plants [ 36 ]. Interestingly, the vibration of the flowers depended on the presence of petals, as flowers that had their petals removed or flowers covered by glass ceased to show a response to the sound vibrations.
3.3. Interpreting Relevant and Irrelevant Sounds
These examples show the important ecological role that sound can play in a plant’s life. Plants don’t live isolated from the rest of the world. Instead, there are extensive connections with other plants, animals, and microbes. Around plants, there are rich communities of arthropods, many of which use vibrations to find mates or prey. For example, vibrations caused by the chewing of Plathypena scabra worms caused predatory Podisus maculiventris stinkbugs to begin their search [ 37 ]. Chewing herbivores produce specific high-amplitude vibrations that travel quickly to other parts of the plant, and this can produce a local and systemic response in other parts of the plant. Arabidopsis thaliana leaves exposed to recordings of caterpillars chewing were proved to be primed for defense [ 38 ]. The plants that had been exposed to chewing vibrations showed higher levels of glucosinolates and anthocyanins following herbivory, while there was no increase in anthocyanins in the plants that either received no vibrations or received vibrations from recordings of leafhopper singing or recordings of the wind [ 38 ]. Interestingly, as with the greater amplitude of bee buzzing increasing pollen removal, higher amplitudes induced higher amounts of aliphatic glucosinolates [ 38 ]. It is still to be understood how the response caused by the vibrations of a herbivore can generate an induced resistance or a systemic resistance (for an in-depth study, see [ 38 ]). One possibility is that the plant subject to herbivory integrates the vibrational signal with others coming from the herbivore’s attack. As plants perceive warning signals via VOCs from nearby stressed plants, and VOCs can serve as a sort of chemical language in the communication between plants [ 39 ], also vibrations can be used at least in some cases in plant communication [ 24 , 40 , 41 ]. This is yet another example of the ecological role that sound can play in a plant’s life. The fact that plants perceive sound from so many different sources and adapt proves them to be ingenuously aware of their environment.
4. Sound below Ground
Sound travels easily and far in dense substrates, and soil is a wonderful example. Since the epigeal part of plants does respond to sound, it might be strange that the roots of plants would not be able to do the same, especially considering that vibrations in the soil are present at all times and places. A possible example of the hypogeal part of plants responding to sound vibrations could be the frequency selective behavioral response of Zea mays roots. When exposed to a continuous sound, the root tips very clearly bend towards the source of the sound [ 42 ]. Furthermore, the root tips very clearly show different responses to different frequencies, with the biggest response elicited by a continuous sound of frequency between 200–300 Hz. Interestingly, the root tips generated acoustic emissions, which could be measured at some distance in the hydroponic medium in which the roots were growing [ 42 ].
Pisum sativum roots showed a behavioral response to sound. Even in the absence of moisture, the roots of P. sativum were able to locate water thanks to the vibrations induced by the movement of the water [ 6 ]. Interestingly, in the presence of moisture, the authors showed that the roots preferred the moisture over the acoustic emissions, suggesting that plants could use the sound of water flowing to locate water and then more accurately find the water using moisture gradients. Interestingly, the roots showed avoidance behavior when in the presence of sound equipment, even when the sound equipment was broadcasting the sound of water flowing. The authors hypothesized that the roots were able to sense a cue, such as magnets in the speakers, that directed their growth away from the sound equipment [ 6 ].
5. Can We Communicate with Plants by Means of Sound
Very clearly, sound has a very important ecological role in the lives of plants, but sound vibration treatment can also be used commercially. For example, treatment of harvested tomatoes with sound was shown to delay ripening. Mature green tomatoes were treated with sound waves of 250, 500, 800, 1000, and 1500 Hz for 6 h. All of the sound treatments except the 800 Hz and the 1.5 kHz delayed tomato ripening, with the 1 kHz treatment having the biggest effect. Seven days after treatment with the 1 kHz sound wave, 85% of the treated tomatoes were still green, whereas over 50% of the non-treated tomatoes had turned red [ 43 ].
The treatment with sound waves was shown to decrease both ethylene production in the treated tomatoes and the respiration rate. By the time the respiration rate of the non-treated tomatoes had begun falling after the completion of the ripening process, the respiration rate in the treated tomatoes was still increasing, suggesting that ripening was indeed delayed. Furthermore, the change in color from green to red was more gradual in treated tomatoes compared with non-treated tomatoes. Finally, the flesh firmness of treated tomatoes decreased more gradually, whereas the flesh firmness of the non-treated tomatoes dropped sharply after five days [ 43 ]. This last result could possibly be explained with the help of a previous study which found that treatment with sound waves decreased the deformability of plant cell membranes and made them more rigid. The sound waves seemed to have an effect not on the cell membranes themselves but seemed to cause microfilaments to rearrange and become more rigid. Interestingly, different frequencies had different effects on the deformability of the cell membranes, with higher frequencies causing the deformability to decrease [ 44 ]. The possibility of delaying ripening through sound wave treatment has important ramifications for lengthening the shelf life of products such as tomatoes.
Treatment with sound can also act as a plant growth stimulant, although the underlying mechanisms for this increase in growth have not yet been properly identified. A possible explanation for this is the fact that sound treatment alters plant growth regulatory hormone levels. Sound treatment increases IAA and decreases ABA levels, and this could be a factor in promoting plant growth [ 10 ]. It was found, for example, that following treatment of Chrysanthemum cells with 1000 Hz, 100 dB sound soluble protein content increased significantly compared with a control group. The treatment lasted for 60 min each day, and the treated plants were separated into groups treated for 3, 6, 9, 12, and 15 days. A rich content of soluble proteins is the basis for many physiological activities. Interestingly, soluble protein content increased significantly after six days and even more significantly after nine days but actually dropped back down when the treatment carried on too long, such as the 12- and 15-day treatments. Furthermore, sugar content increased following stimulation compared with the control group. Finally, amylase activity increased following stimulation compared with the control group. The importance of frequency in the response of plants to sound is shown time and time again. While 1000 Hz sound was shown to be beneficial by increasing soluble protein and sugar content and increasing amylase activity, 2000 Hz sound actually proved to be damaging for plant cells [ 19 ].
Other examples of sound treatment as a growth stimulant could be the increased yields in sound-treated tomato or the treatment of wheat with sound waves of 92 dB and 5 kHz to increase yield and dry weight. Photosynthesis was shown to increase following sound treatment in rice and strawberries, and photosynthesis-related proteins were highly expressed following 8-h sound treatments at 250 or 500 Hz in Arabidopsis thaliana [ 10 ]. Sound increased the resistance of strawberries to insects and disease [ 23 ]. SV treatment brought about an increase in the length, number, and activity of Actinidia chinensis roots. Highly dormant seeds of Echinacea Angustifolia showed enhanced germination following treatment with sound vibrations of 1000 Hz and 100 dB [ 23 ]. SV treatment has possible applications in biotechnology, ultrasound being able to enhance Agrobacterium-mediated transformation of several plant species, or audible SVs showing to increase in vitro growth of many plant species [ 45 , 46 , 47 , 48 , 49 , 50 ].
Sound treatment was also shown to induce drought tolerance in Arabidopsis thaliana , leading to a significant increase in survival rates compared with control plants [ 51 ]. At the end of the treatment, plants were sampled to determine changes in transcription. Eighty-nine genes were found to have had their expression altered, 87 of which upregulated, the remaining two downregulated. Of the 87 upregulated genes, 44 are involved in stress-related responses [ 51 ].
The Case of Cavitation
Considering that plants do respond to sound, could plants themselves actually emit sounds? Going even further, if plants do emit sounds, could other plants, or perhaps the same plant, perceive these acoustic emissions and react to them? As mentioned previously, it was indeed found that corn roots grown hydroponically emitted sounds [ 42 ]. However, prior to this discovery, it was already believed that in conditions of drought, cavitation in xylem vessels could be a source of acoustic emissions [ 9 ]. Cavitation is the mechanical breakage of the continuous water column in a xylem vessel that occurs when the tensile strength of the water column is exceeded. This is accompanied by the build-up of mechanical pressure, which, when released, leads to elastic wave propagation [ 11 ]. In other words, there is an abrupt release of tension in the xylem vessel lumen as the liquid water under negative pressure is replaced by water vapor [ 52 ].
Previously the measurement of acoustic emissions following cavitation was done through actual contact between sensors and the plant itself [ 53 ]. Although still interesting, this method does not take into account whether these emissions could be sensed at a distance. However, plants do emit airborne sounds that can be detected from a distance. Different tomato ( Solanum Lycopersicum ) and tobacco ( Nicotiana tabacum ) plants were placed in an acoustically isolated anechoic box under different treatments and were recorded simultaneously at a distance of 10 cm by two directional microphones in order to eliminate false detections of clicks caused by the electrical equipment. The plants were either cut, placed under drought stress, or were in control conditions. The plants that were under stress or cut emitted significantly more sounds compared with the control plants. For the drought-stressed plants, the mean number of sounds emitted per hour was 35.4 for tomato and 11.0 for tobacco, while for cut plants, the mean number of sounds emitted per hour was 25.2 and 15.2 for tomato and tobacco, respectively. Surprisingly, the control plants not subjected to either drought or cutting emitted less than one sound per hour [ 53 ].
Although the precise values differ slightly for tomato and tobacco, the mean peak frequencies of the emitted sounds, in other words, the frequencies with the maximal energy, were between 49 kHz and 58 kHz. These results not only indicate that the emitted sounds are ultrasonic, that is above 20 kHz and not detectable by the human ear, but also confirm that these emissions are detectable at least at a distance of 10 cm. This means that these emissions could be theoretically detected by other organisms, such as insects or other plants [ 53 ].
What makes this experiment especially interesting is the use of machine learning, which refers to a system’s ability to improve and extend itself by learning new knowledge rather than being programmed with that knowledge [ 54 ], to determine whether it was possible to identify the condition of a plant based on the sounds it emitted. The regularized machine learning classifier, which was also trained to discriminate against the electrical noises made by the recording equipment, was able to correctly identify the condition of the plants based on the sounds they emitted. Not only could it distinguish between the control plants and the treated plants, but it could also distinguish between the cut plants and the drought-stressed plants. This is fascinating because it could mean that the sounds that plants emit when under drought stress could carry information; therefore these sounds could be intercepted by other organisms who could then respond and adapt [ 53 ]. Finally, tomato plants were placed in a greenhouse to simulate more realistic conditions [ 53 ]. The recording equipment was trained to discriminate between tomato sounds and greenhouse sounds. A consistent acoustic pattern was found in that the number of sounds emitted is very low when the plant has been recently irrigated, but the number of emissions drops as the plant becomes dry.
These ultrasound emissions could be detected at a distance of 3 to 5 m. This means that it is possible that these acoustic emissions could be perceived by other organisms. For example, many moths that use tomato and tobacco plants as hosts for their larvae can perceive sounds in the frequencies and intensities that were detected in this experiment. It is possible that the information contained in the acoustic emissions of drought-stressed plants could inform these moths not to lay their eggs on these plants [ 53 ]. The emission of sound by drought-stressed plants has important implications in agriculture, as the detection of these sounds could be used to monitor the water status of crops, and this could, in turn, lead to more efficient and precise irrigation, therefore reducing water usage.
Interestingly, it seems that the acoustic emissions of plants can actually be distinguished between low-dB Ultrasonic Acoustic Emissions (UAE), which are below 27 dB, and high-dB UAE (above 35 dB) associated with cavitation. Most investigations on acoustic emission detection have focused on higher dB ranges under the assumption that low dB sounds cannot be distinguished from background noise. However, it was found that signals in the low dB range seem to have a consistent pattern. UAE remained in the low dB range on sunless days and at night and transitioned abruptly to the high dB range on sunny days. High dB acoustic emissions coincided sharply with decreased sap flow rate in Quercus pubescens [ 52 ].
Typically, low dB acoustic emissions increase in intensity as bark tissue expands with hydration, so at night or while it is raining, the intensity of the low dB emissions increases. During the day, as transpiration occurs, the diameter of the stem shrinks as water is lost, and the intensity of the low dB emissions decreases gradually until there is a very abrupt transition to the high dB emissions that are probably caused by cavitation. The highest low dB acoustic emissions occur before dawn, which is when the least water movement occurs. So low dB acoustic emissions closely follow stem radius changes de-trended for growth. There are different possible origins for the low dB emissions [ 52 ].
One possible source for the low dB sound could be the mechanical noise of the stem shrinking and expanding. Or it could be the respiration and metabolic growth activity of the cambium and ray parenchyma cells. These obviously produce diurnal courses of CO 2 efflux from the stem. If the water content is high enough, then the respiration rate follows the temperature. At low water contents, however, the missing water seems to inhibit biochemical activity, regardless of temperature. In conditions of drought, respiration follows stem water content more closely and is largely independent of temperature. Under such conditions, low dB acoustic emissions and stem water content match respiration. When the turgor pressure in the cambium increases, for example, at night, radial growth occurs; consequently, respiration increases, and low dB acoustic emissions increase [ 52 ].
For a long time, it was thought that sounds generated by plants were always a product of cavitation, but the overabundance of sound emissions by plants makes it highly unlikely that all sounds generated by plants are a product of cavitation, considering the limited number of water-conducting elements. Although it seems clear that cavitation can indeed emit sound, some authors believe that sounds generated from the xylem area are not caused by cavitation but by a stable bubble system capable of transporting water through peristaltic waves. Laschimke and colleagues believe that acoustic emissions are a result of sudden surface rearrangements of groups of wall-adherent microbubbles under positive pressure. These microbubbles, which have also been photographed, are largely stable and do not immediately result in embolism [ 55 ]. Laschimke also found, in Ulmus glabra , that acoustic emissions are incessant during transpiration and re-hydration. Therefore it is very unlikely that all acoustic emissions are a result of cavitation. Acoustic activity is undiminished during the night. This means that acoustic activity is not solely a result of transpiration, although transpiration does modify the type of activity, as stated before. The authors analyzed the waveforms of the acoustic emissions in U. glabra during a testing period of 77 h. By analyzing the waveform, it is possible to better understand the underlying physiological processes that cause the acoustic emission [ 56 ]. It is reasonable to expect that a sound emitted by a cavitation event would have a very rapid fading of the acoustic signal, as the water column is rapidly and violently retracted along the vessel following the disruption. However, very few of the 2200 acoustic emission events had a waveform profile of this type. Instead, most acoustic signals showed great variability in the duration, amplitude, and frequency, which can hardly be explained by the cavitation theory of acoustic emission.
6. Plant Alerts
Communicating drought stress.
At this point, it is clear that not only do plants respond to sound, but also that plants emit a wide variety of acoustic emissions, with varying frequencies, from audible to ultrasound, and varying durations and intensities. However, it is harder to actually pinpoint the source of these emissions, and the theory that acoustic emission was a result of cavitation has been put into question, or at least it has been shown that not all emission is a result of it.
As stated before, sound treatment increased drought tolerance in A. thaliana . The same was shown in Oryza sativa . Different rice plants were treated with single frequencies of 0.25, 0.5, 0.8, 1.0, and 1.5 kHz for 24 h. After this treatment, the plants were placed under drought stress for five days. Sound treatment with frequencies of 0.8 kHz and above increased stomatal conductance, relative water content, and quantum yield of PS II. Furthermore, hydrogen peroxide production was inferior in these plants, and the temperature of the sound-treated plants and leaves was inferior compared with control [ 57 ]. So, could it be possible that the acoustic emissions by plants could be perceived by other plants? It has already been mentioned that certain moths can detect sound in the frequencies emitted by drought-stressed tomato and tobacco plants and possibly avoid laying their eggs on those stressed plants; a machine learning tool could very clearly distinguish between stressed and control plants. Could a drought-stressed plant emitting cavitation sounds, among other sounds, alert other plants of impending drought stress? This is a possibility.
Freeze-thaw cycles are the second most important reason for inducing cavitation, so it was natural for studies to focus on the acoustic emission of plants following such cycles. A study found that ultrasonic acoustic emissions are detected during the freezing part of the cycle in conifers, occurring during the ice formation part of the cycle, and most UAE are perceived during the first freeze-thaw cycle, with lower emissions during subsequent cycles. It was also found that samples with water contents close to dehydration emitted UAE during temperature cycles, whereas very dehydrated samples or saturated samples showed few UAE [ 58 ].
But why should plants communicate through the use of sound? What possible advantages could be obtained through the use of sound, as opposed to the use, for example, of VOCs? Firstly, physical signals such as sound can propagate very rapidly, as opposed to VOCs that need to diffuse through the air. Moreover, sounds can be analyzed quickly and can be sensed at very low intensities and over long distances. Sound not only propagates a lot faster than volatiles, but it also has the added benefit of allowing for more accurate source localization. This means that sound has features that degrade predictably over distance, allowing a receiver to estimate the distance from the emitter. Not only that, but acoustic emission is also the result of a physical process, at least in the case of cavitation, which means that there is little to no energy cost involved [ 24 ]. VOCs, on the other hand, represents a significant loss of energy, and a substantial amount of the carbon fixed by plants is re-emitted into the atmosphere through VOC communication [ 59 ]. A possible advantage of VOCs, however, could be their ability to linger in their environment after emission, whereas acoustic signals very obviously dissipate extremely quickly. However, it’s also important to note that volatile signals depend on diffusion and wind direction and, therefore, also suffer from their dilution. This means that although VOCs can linger, they nevertheless need to be present in sufficient quantities to be able to be perceived. Sound, on the other hand, can be perceived by organisms even at very low intensities [ 24 ].
7. Conclusions
At this point, it should be clear that plants respond and emit sound in a wide variety of intensities, frequencies, and durations, as a result of different mechanisms. This is unsurprising in a way, considering the age of plants on Earth and the omnipresent distribution of sound. More study on the acoustic emission of plants is needed to properly understand just what causes sound in plants and how many possible sources of sound there are. A better understanding of sound emission could also clarify other mechanisms, such as cavitation following freeze-thaw cycles and, in general, what happens inside a plant during stress in a non-invasive and real-time manner.
A common theme in studies focusing on the role of sound in plants is the scarce knowledge of the molecular and cellular mechanisms of sound perception and signal transduction. More research at this level of the plant system could determine just how plants react and produce sound, and this could help clarify the ecological role of sound communication in plants. After all, specialized receptors or proteins involved solely in sound perception have yet to be identified in plants. Further research can also clarify the differences and similarities, if they exist, between sound and mechanical stimulation. After that, considering the similarities in essence, the propagation, and in the effects between touch and sound, could light mechanical stimulation cause the same reactions as sound treatment?
Studies on sound perception in plants need to continue also because they have important implications in agriculture and biotechnology. Being able to accurately assess the water status of crops through their acoustic emissions can lead to more efficient irrigation, for example. Sound can also be used to increase the shelf life of products, increase yields, or to trigger plant defenses against pathogens. However, if sound treatment of plants begins widespread use, it is also important to consider its potential side effects on animals, humans, and plants. After all, realizing that plants respond to sound means also accepting that noise pollution affects the plant world as well. Sound treatment on plants needs to be wary of increasing noise pollution problems.
Plants have long been considered to be unchanging, passive, and static organisms, but this view needs to change. Plants are far more ingenious and aware than initially thought, and changing the way we view plants can lead to research that can better take into consideration their capabilities.
Funding Statement
This research received no external funding.
Author Contributions
Conceptualization, L.A.; writing—original draft preparation, F.D.S.; writing—review and editing, V.M.; supervision, L.A. and L.F. All authors have read and agreed to the published version of the manuscript.
Data Availability Statement
Conflicts of interest.
The authors declare no conflict of interest.
Publisher’s Note: MDPI stays neutral with regard to jurisdictional claims in published maps and institutional affiliations.

IMAGES
VIDEO
COMMENTS
2.1. Eligibility Criteria. The eligibility criteria for inclusion of a study in this research were as follows: (1) participants of any type were recruited; (2) no criteria were set for the type of indoor plant to be used in interventions; (3) the comparator was participants in an indoor environment without any plants or with other elements; (4) the outcome included any type of objectively ...
Plant sciences is the study of plants in all their forms and interactions using a scientific approach. ... Research Open Access 10 Apr 2024 Nature Communications. Volume: 15, P: 3122.
Experimental research and field studies on plants (either cultivated or wild), including the collection of plant material have complied with relevant institutional, national, and international ...
Studies have been carried out globally to verify their efficacy and some of the findings have led to the production of plant-based medicines. ... Olatunji BP, Adesoga AA. Ethno medicinal information on collation and identification of some medicinal plants in Research Institutes of South-west Nigeria. African Journal of Pharmacy and Pharmacology ...
This study by Scacchi et al. shows that a mobile small-RNA-based Turing system dynamically organizes plant organ polarity. The afforded developmental flexibility accounts for diversity in organ ...
Summary. Plants provide us with oxygen, food, fuel and fiber. Among other reasons, scientists study plants to improve and secure the food supply for an increasing world population, identify new sources of bioactive compounds and medicines, improve fiber production and identify sources of biofuels and biorenewable resources.
Looking ahead, fundamental research on model organisms, applied work on crops, and conservation studies on rare plants will all continue to be of vital importance to modern plant biology. High-throughput inquiries and gene-specific projects done by mega-groups and small labs in state-of-the-art facilities or traditional field labs will all ...
Advances and Horizons in Plant Molecular Farming: Pathways to Sustainable Biopharmaceuticals and Beyond. Kevin Yueju Wang. Yongfeng Guo. 308 views. The most cited plant science journal advances our understanding of plant biology for sustainable food security, functional ecosystems and human health.
The history of the relationship between plant growth and water consumption is retraced by following the progression of scientific thought through the centuries: from a purely philosophical question, to conceptual and methodological developments, towards a research interest in plant functioning and the interaction with the environment. The relationship between plant growth and water consumption ...
As plant science becomes increasingly important, we need to attract the brightest and best to careers in plant research. School education does not include the most interesting or relevant aspects of plant science, and discourages young people from studying the subject at university. This is indefensible in a world with such a strong requirement ...
This study supports previous research which showed that indoor plants positively affected people's perceptions of IAQ and environmental quality [17, 25], but it also advances this area of knowledge by showing that these perceptions and the extent of the perceived benefits are affected by the appearance of the plant. 3.9.
The rapid extinction of endangered plants (EPs) may lead to the destruction of entire ecosystems, which will seriously threaten the survival and development of humans. Research on endangered plants should be strengthened to scientifically guide the protection of endangered plants. Based on 1635 publications collected from the Web of Science Core Collection™ (WoS), this paper aims to provide ...
In addition, scientists can investigate how plants respond to reduced gravity environments on the moon and Mars. This researcher's guide provides information on the background for plant science research, summarizes previous research, including lessons learned, and provides information on ISS research facilities.
Plant physiology is a sub-discipline of botany concerned with the physical, chemical and biological functioning of plants. Latest Research and Reviews Comparative studies of four cumin landraces ...
Plant Growth. Plants are vital to all life on Earth. They are important because plants take in carbon dioxide from the atmosphere and produce oxygen. In addition, plants make up the base of the food web by producing their own food using light, water, carbon dioxide, and other chemicals. This is why they are known as producers or Autotrophs.
botany, branch of biology that deals with the study of plants, including their structure, properties, and biochemical processes. Also included are plant classification and the study of plant diseases and of interactions with the environment. The principles and findings of botany have provided the base for such applied sciences as agriculture ...
Benjamin Cole, senior author of the Nature Plants paper describing one of the first cross-kingdom spatially-resolved transcriptomics studies to date. For millions of years, underground fungi have ...
According to a 2010 study conducted by the Royal Botanic Gardens in the UK, 22% of the approximately 380,000 known plant species (or about 83,600 plant species ... cutting edge research. Plants will entertain original research articles, mini reviews, reviews, communications, short notes and suggestions for special and focused issues in the ...
The objective of this research was to assess the effects of irrigation rate on plant growth and fruit yield in eggplant. The study was conducted in Tifton, GA, in the fall of 2010 and 2011. Eggplant plants cv. Santana were grown on raised beds (1.8 m centers) covered with white plastic film mulch.
In recent years, there have been several studies regarding the benefits of plants. The findings of these studies have uncovered an impressive array of advantages to being a plant parent. 1. Plants May Help to Reduce Stress Levels. It's no secret that our modern world is becoming more stressful than ever before.
Adult-plant resistance showed 153 (96.2%) germplasms represented resistance in 2022, while only 85 (53.4%) showed resistance in 2023. An association study using the 55K SNP chip data results combined with disease ratings of 159 materials at both the seedling and adult stages discovered 593 loci related to stripe rust resistance (P ≤ 0.0001).
In eukaryotic organisms, proper chromosome segregation during cell division depends on the centromeric histone H3 (CENH3) variant. Our previous studies identified a plant CENH3 assembly factor, Kinetochore Null2 (αKNL2), that possesses a centromere-targeting motif, CENPC-k, similar to the CENPC motif in CENP-C. Additionally, we have demonstrated that αKNL2 can bind DNA in vitro, independent ...
Plant function responses vary between −28% and 29% (5th to 95th percentile) under fewer, larger rainfall events, with the direction of response contingent on climate; productivity increases are ...
The experiment is open to all, requiring only a small, sunny place for plants to grow from each citi-scientist. If selected for the research project, participants will receive plant pickup dates, location details and more information with additional resources. Contact Gomez at [email protected] or 765-494-4443 for any questions.
To transfer experimental findings in plant research to natural ecosystems it is imperative to reach near to natural-like plant performance. Previous studies propose differences in temperature and light quantity as main sources of deviations between indoor and outdoor plant growth. ... (30% B and 70% R) in the current study, plants under 63% B ...
The expert plant science and space technology partners come from UWA and the universities of Cambridge, Adelaide and Southern Queensland and work with industry partners Axiom Space (US), and Saber Astronautics (USA and Australia). UWA International Space Centre is hosting a Plants in Space free public lecture on Wednesday April 10 at 1pm.
Research has uncovered important new insights into the evolution of oxygen, carbon, and other vital elements over the entire history of Earth -- and it could help assess which other planets can ...
Dr. Culley is a professor of plant biology at the University of Cincinnati. She led UC's long-term study on the UC Harris Benedict Nature Preserve that was damaged in that storm. ... Those studies ...
A growing body of research is showing that plants detect and emit sounds. This is unsurprising considering that there is no habitat colonized by plants that is without sound and taking into account their sessile nature and their age on Earth, it is reasonable to think that they have learned to do so. 2.1.